Temperature Management in Cardiac Surgery
Laurie K. Davies
Heather Reed
The use of hypothermia as an adjunct to the treatment of a wide variety of disorders has been advocated for centuries. Lowered body temperature has been employed to combat cancer, infection, trauma, and central nervous system diseases, and as a regional method to induce anesthesia for amputation (1,2). However, it was not until 1950 that Bigelow et al. (3) demonstrated longer tolerance to inflow occlusion in hypothermic animals than in their normothermic counterparts. This work led to the first clinical application of hypothermia in cardiac surgery. Lewis and Taufic (4) used surface cooling to 28°C with 5.5 minutes of inflow occlusion to facilitate successful closure of an atrial septal defect in a 5-year-old child. In 1952, Gibbon (5) introduced the pump oxygenator to clinical practice, and in 1958, Sealy et al. (6) used hypothermia in conjunction with the cardiopulmonary bypass (CPB) circuit for intracardiac repairs. The use of the pump oxygenator and hypothermia has allowed cardiac surgery to flourish. Complex lesions are repaired routinely with remarkably low mortality. However, it is now recognized that inadvertent hyperthermia occurs relatively frequently in the perioperative setting. Evidence is accumulating that elevated temperatures may be deleterious in both animals and humans (7,8). A better understanding of the principles of temperature management may maximize the benefits to our patients while minimizing potential complications.
PHYSIOLOGY OF HYPOTHERMIA
One of the main difficulties in devising a reasonable strategy for the application of hypothermia in humans is the fact that they are naturally homeothermic beings. Humans and other homeothermic species have very effective homeostatic systems, which ensure that the body temperature remains consistently near 37°C regardless of changes in environmental temperatures. This tight regulation of temperature is accomplished by multiple mechanisms. Cold is sensed by the thermoreceptors in the skin, which then causes the hypothalamus to trigger a strong sympathetic nervous system response. Vasoconstriction of skin vessels, which decreases convective heat loss, occurs simultaneously with vasodilation of the skeletal muscle vascular beds, which augments muscular activity to produce heat by tensing and shivering. The endocrine system is activated, oxygen consumption is increased, and heart rate, cardiac output, and blood pressure are elevated. Because of the complexity of these interactions, one can appreciate the difficulty in understanding the physiologically appropriate response to the unnatural state of induced hypothermia in humans. One must extrapolate from animal studies, biochemical equations, accidental hypothermia survivors, and normal organ temperature gradients to try and develop the most effective management strategy when using deliberate hypothermia.
Rationale for the Use of Hypothermia
Why is hypothermia often employed during CPB? The obvious purpose of using hypothermia is to provide a degree of organ (and organism) protection and safety margin during CPB. Hypothermia exerts its protective effect by multiple mechanisms. The most obvious mechanism is a reduction in metabolic rate and oxygen consumption (9) (Fig. 8.1). This metabolic suppression may not explain all the protective effects observed. Hypothermia also helps preserve high-energy phosphate stores and reduces excitatory neurotransmitter release, which is especially important to central nervous system protection (10,11). Normally, ischemic neuronal cells rapidly release neuroexcitatory amines, especially glutamate (12). The accumulation of these excitotoxic amines causes the opening of calcium channels and activation of multiple destructive enzymatic systems. Hypothermia attenuates this excitotoxic cascade, helping to prevent calcium entry into the cell and restricting membrane permeability (13) (Fig. 8.2).
Alteration of temperature causes a change in the reaction rate of all biochemical processes, especially enzymatic reactions. This temperature dependence of reaction rates has been described by the concept of Q10, which is defined as the increase or decrease in reaction rates or metabolic processes in relation to a temperature change of 10°C. For instance, the reaction rate of a process with a Q10 of 2 will double with a 10°C increase in temperature or be halved with a drop of 10°C. Most reactions, including total body oxygen consumption, have a Q10 of 2 to 3 (9).
Some biochemical processes, especially those localized to cell membranes, show an abrupt change in reaction rates at certain critical temperatures. This has been termed a phase
transition and is thought to be the result of a change in the cell membrane from a fluid to a gel state (14). In mammalian tissues, phase transitions often occur at approximately 25°C to 28°C and may disturb cell homeostasis. Biophysical processes such as osmosis and water diffusion are also affected by temperature. Typically, a linear change of approximately 3%/10°C is seen. Therefore, this effect is minimal at clinical levels of hypothermia. However, if the freezing point of water is approached, ice is formed in the tissue, a condition that is not tolerated. The solutes concentrate in a hyperosmolar manner in the residual nonfrozen water, causing marked fluid shifts and membrane disruption. Mammalian tissue does not regain function on thawing from a frozen state. For this reason, there is a limit to the beneficial effects of hypothermia.
transition and is thought to be the result of a change in the cell membrane from a fluid to a gel state (14). In mammalian tissues, phase transitions often occur at approximately 25°C to 28°C and may disturb cell homeostasis. Biophysical processes such as osmosis and water diffusion are also affected by temperature. Typically, a linear change of approximately 3%/10°C is seen. Therefore, this effect is minimal at clinical levels of hypothermia. However, if the freezing point of water is approached, ice is formed in the tissue, a condition that is not tolerated. The solutes concentrate in a hyperosmolar manner in the residual nonfrozen water, causing marked fluid shifts and membrane disruption. Mammalian tissue does not regain function on thawing from a frozen state. For this reason, there is a limit to the beneficial effects of hypothermia.
In cardiac surgery, CPB in conjunction with systemic hypothermia allows lower pump flows, better myocardial protection, less blood trauma, and better organ protection than does normothermic perfusion (15). Oxygen needs predictably fall with lowered temperature. It was recognized early that lowered bypass flows could be employed in this setting and still provide adequate perfusion, as assessed by mixed venous oxygen tension and return of organ function following bypass. Relating oxygen consumption (VO2) to perfusion flow rate at various temperatures can also be valuable in assessing adequacy of tissue perfusion (Fig. 8.3). At a given temperature, a fall in VO2 with a decrease in flow rate implies a flow-limited VO2, indicating that oxygen delivery is not adequate. Hickey and Hoar (16) have shown in humans that a reduction in flow rate from 2.1 to 1.2 L/min/m2 of body surface area at 25°C does not alter VO2 or tissue perfusion. Slogoff et al. (17) were unable to correlate low flows (<40 mL/kg/min) or pressures (<50 mm Hg) during bypass in which moderate hypothermia and hemodilution were used with postoperative renal or central nervous system dysfunction. Lower perfusion flow rates allow better visualization by the surgeon. Venous return from the bronchial, pulmonary, and noncoronary collateral vessels is also decreased. Because this returning blood is at systemic temperature, it can inappropriately warm the heart when cardioplegia-induced myocardial hypothermia is at a lessthan-systemic temperature and can jeopardize myocardial protection. Blood trauma is minimized because of both the lower pump flows and the hemodilution employed during bypass. Because the etiology of most central nervous system damage on bypass may be embolic in origin (18), lower bypass flows can minimize these focal insults. Systemic hypothermia also provides some margin of safety for organ protection if equipment failure occurs or circulatory arrest must be employed.
Acid-Base Alteration with Temperature Change
One of the more often discussed aspects of clinical hypothermia is the appropriate acid-base management strategy during hypothermia. To understand acid-base regulation during hypothermia, it is helpful to consider several example conditions. “Normal” values for pH and Pco2 are usually thought of as 7.40 pH units and 40 mm Hg, respectively. However, it must be kept in mind that these values are appropriate only at 37°C in blood. There is a temperature-dependent spectrum of “normal” values, depending on the temperature of the specific site in the body. For example, arterial blood leaves the heart at 37°C with a pH of 7.40 and a Pco2 of 40 mm Hg. When that same blood perfuses working skeletal muscle, where the ambient temperature may be 40°C, it will have a pH of approximately 7.35 and a somewhat higher Pco2, despite a constant CO2 content before any respiratory exchange with the muscle. In contrast, the same arterial blood perfusing exposed skin, where temperature may be 20°C in cool weather, will have a pH of 7.65 and a proportionately lower Pco2, again with no change in CO2 content. Stated another way, a sample of arterial blood held in a gas-tight syringe will have a Pco2 that varies directly with temperature, and a pH that varies inversely with temperature, despite constant CO2 content.
This change in Pco2 with temperature is a consequence of the change in solubility of gases in liquids with change in temperature. As a general rule, decreasing the temperature of a liquid increases the solubility of a given gas in that liquid and therefore decreases the partial pressure of that gas while the overall content of the gas in the liquid remains constant. Increasing the temperature of a liquid increases the kinetic energy of the molecules in the liquid, which both increases the tendency of dissolved gas molecules to leave the liquid (decreased solubility) and increases the partial pressure of those gas molecules remaining in the liquid. This concept is intuitive to anyone who has opened a container of warm carbonated beverage and observed that its tendency to “fizz” (CO2 bubbling out of solution) is much greater than that of a cold beverage.
The relation of pH to temperature is somewhat more complex than the change in Pco2 with temperature. There is clearly a direct relation between the concentration of H+ [H+]
in a water solution and the CO2 content of that solution. The higher the CO2, the more H+ in solution, and hence lower the pH. This is largely caused by the tendency of CO2 to combine chemically with water to produce carbonic acid, which then dissociates in solution to yield H+. Accordingly, one might expect that the change in pH of blood with temperature is caused by the changes in Pco2 with temperature, as discussed in the preceding text. It is true that cooling an anaerobic blood sample decreases both the Pco2 and the [H+]. However, and very importantly, the change in [H+] and pH that occurs with change in temperature is independent of a change in CO2 content, and therefore does not depend on the change in CO2 solubility with the temperature change.
in a water solution and the CO2 content of that solution. The higher the CO2, the more H+ in solution, and hence lower the pH. This is largely caused by the tendency of CO2 to combine chemically with water to produce carbonic acid, which then dissociates in solution to yield H+. Accordingly, one might expect that the change in pH of blood with temperature is caused by the changes in Pco2 with temperature, as discussed in the preceding text. It is true that cooling an anaerobic blood sample decreases both the Pco2 and the [H+]. However, and very importantly, the change in [H+] and pH that occurs with change in temperature is independent of a change in CO2 content, and therefore does not depend on the change in CO2 solubility with the temperature change.
All acids and bases, including water, exist in solution in equilibrium between the undissociated form and the ionized components of the parent molecule. The dissociation constant (K) is the equilibrium ratio of the product of the concentrations of the ionized components to the unionized component. For water at 25°C, the equilibrium dissociation equation is as follows:
K(H2O, 25°C) = [H+] × [OH–]/[H2O] = 1.08 × 10-14
Because, at equilibrium in water, [H+] equals [OH–], and because the concentration of H2O is essentially 1, [H+] equals [OH–] equals [check mark]10-14, or 10-7. Because the definition of pH is the negative log10 of [H+], the pH of pure water at equilibrium at 25°C is 7. This pH value has come to be called the neutral pH, or pN, of water. Temperature change has a significant effect on the tendency of molecules in solution to dissociate. In thermodynamic terms, the increased kinetic energy associated with increased temperature promotes dissociation, whereas decreased temperature has the opposite effect. For example, within the temperature range seen in clinical CPB (approximately 15°C-40°C), the dissociation constant of water increases from 0.451 × 10-14 to 2.919 × 10-14. These values of KH2O relate to a change in [H+] from approximately 67 nmol/L at 15°C to 170 nmol/L at 40°C. This nearly 3-fold change in [H+] is solely a consequence of the effects of temperature change on dissociation. Pure water may be considered the simplest weak acid-base solution. The major importance of these concepts is that water is the fundamental solvent of all biologic systems, and the dissociation of virtually all weak acids and bases in biologic solutions follows the same pattern as that described for water.
The behavior of body fluids (intracellular and extracellular, intravascular and extravascular) is far more complex than the simple scenario described earlier for water, but biologic fluids behave much like water in terms of the intrinsic temperature-related changes in the dissociation constants of the many weak acids and weak bases, of which they are composed. The amino acids in proteins, the simple sugars in polysaccharides, the fatty acids in lipids, and the major buffer systems, all follow this same basic pattern. As the temperature decreases, the tendency to dissociate decreases, and the concentrations of the ionized components (H+ and R–) also decrease.
At normal body temperature (37°C), blood and tissue fluids are alkaline (lower [H+] and correspondingly higher pH) relative to water at the same temperature. A number of buffer systems create and maintain this relative alkalinity so that the ratio of [OH–] to [H+] remains constant at approximately 16:1 despite temperature variation. As temperature changes, the intrinsic dissociation of these buffer systems also changes to maintain the ratio of [OH–] to [H+] constant. Therefore, the intrinsic pH shift of blood and tissue fluid parallels the pNH2O as temperature changes, and this relative alkalinity remains constant in comparison with water (19) (Fig. 8.4).
A major buffering system responsible for this constant relation of blood and tissue fluid pH to pN, with temperature change, is the imidazole moiety of the amino acid histidine, which is commonly found in body proteins. The pKa of this component of histidine is close to 7.0 at body temperature, a property that confers potent buffering capacity for maintaining a constant ratio of [H+] to [OH–] despite significant changes in the absolute concentration of each as temperature varies. These considerations are applicable to the previous example of arterial blood with a constant CO2 content perfusing tissues with different temperatures. The observed shift in pH with cooling follows the pNH2O, and the buffering capacity of the imidazole moiety of histidine preserves the constant relative alkalinity and ratio of [OH–] to [H+] in the blood (Fig. 8.5). In cold-blooded vertebrates, the blood pH-temperature curve also runs parallel to the pH of neutral water. Intracellular pH has also been measured in various animals and shows changes with temperature identical to those that have been described for pNH2O (20) (Fig. 8.6). The intracellular pH parallels the pN and blood pH slopes with temperature changes and differs from the extracellular pH by a constant but species-specific factor of approximately -0.6 to -0.8 pH units. Therefore, at 37°C, intracellular pH is approximately 6.8 to 6.9 and so the [H+] is somewhat higher. In a manner similar to the observed change in blood pH with temperature, the reaction kinetics of numerous respiratory enzyme systems (lactate dehydrogenase, Na+-K+-ATPase [sodium-potassium adenosine triphosphatase], acetyl CoA carboxylase, fatty acid
synthetase, NADH [reduced nicotinamide adenine dinucleotide] cytochrome c reductase, and succinate cytochrome c reductase) all show optimal catalytic function with temperature change when the pH of the reaction medium parallels the temperature-mediated pNH2O change (21).
synthetase, NADH [reduced nicotinamide adenine dinucleotide] cytochrome c reductase, and succinate cytochrome c reductase) all show optimal catalytic function with temperature change when the pH of the reaction medium parallels the temperature-mediated pNH2O change (21).
This constant internal milieu is accomplished, as has been mentioned, predominantly by the buffering capacity of the imidazole group of the amino acid histidine. As temperature changes, the imidazole groups in protein change pKa in parallel with the pN of water. The ratio of the unprotonated histidine imidazole groups to H+, a value known in the world of chemistry as alpha, remains constant, total CO2 also remains constant, and the pH changes as per the changes in temperature. The term α–stat has come to indicate an acid-base management strategy in which the net charge (dissociation) of proteins remains constant as temperature changes. Typically, this is managed during CPB by keeping total CO2 stores constant and allowing pH and PaCO2 to follow their thermodynamically mediated dissociation changes with changes in temperature. In other words, during cooling, exogenous CO2 is not added to the system when following the α-stat strategy.
The alternative method of acid-base strategy is termed pH-stat. With this method, pH is the value that is maintained constant at varying temperatures. Obviously, if the pH-stat strategy is used when blood is cooled, CO2 must be added to maintain a Paco2 of 40 and a pH of 7.40. Extracellular and intracellular ratios of [OH–] to [H+] are altered and the total CO2 stores are elevated.
Why might one strategy be chosen over another? During the first two decades of hypothermic CPB, pH-stat management with the addition of 5% CO2 to the oxygenator gas flow was used almost exclusively. An understanding of the expected changes in pH with temperature seemed to be lacking, and CO2 was thought to be beneficial for cerebral vasodilation and maintenance of cerebral blood flow (CBF). In the last 30 years, this practice has been questioned, and many institutions have shifted toward an α-stat management protocol. It is only recently that sufficient data have accumulated to enable a rational decision to be made for employing the more suitable of the two strategies in a given situation.
On a theoretical basis, α-stat management may be preferable in certain situations. Maintenance of constant intracellular electrochemical neutrality appears to be essential for normal cellular function (22). Intracellular metabolic intermediates of high-energy phosphates can be depleted if there are changes in the intracellular pH and these metabolites lose their charged state. These substrates are then free to diffuse across lipid membranes. Most enzymes depend on optimal pH for their function. Electrochemical neutrality is also important in maintaining the Donnan equilibrium across cellular membranes to allow normal intracellular anion concentrations and water content (23).
Poikilothermic animals, whose tissues must function optimally despite wide variations in temperature, follow an α-stat acid-base strategy. On the other hand, hibernating mammals appear
to maintain a pH-stat strategy, with constant (temperature-corrected) blood pHa and PCO2 (22). These animals hypoventilate as they hibernate, the tissue CO2 stores increase, and intracellular pH becomes acidotic in most tissues. This acidotic state causes a further depression of metabolism that teleologically may be useful by further decreasing the energy consumption of nonfunctioning tissues, such as skeletal muscle, gastrointestinal tract, and higher brain centers. In contrast, active tissues, such as heart and liver, adopt a different strategy by actively extruding H+ across their cell membranes to maintain intracellular pH at or near the values predicted by the α-stat methodology. Therefore, hibernating mammals are able to vary their intracellular-to-extracellular pH gradient differently in different tissues, depending on the state of metabolic activity of the tissue. Functionally, this provides different types of acid-base regulation in different tissues, depending on the metabolic activity of the tissue. The first noticeable change associated with arousal from hibernation is hyperventilation. This depletes the CO2 stores, raises intracellular pH, and increases the metabolic rate. The animal reverts to an overall α-stat pH control pattern during awakening, which allows tissues to regain optimal function. Therefore, in hibernating mammals, the issue of acid-base maintenance is not clearly defined because intracellular acid-base regulation can be independent of blood regulation both within and among different tissues in the same animal.
to maintain a pH-stat strategy, with constant (temperature-corrected) blood pHa and PCO2 (22). These animals hypoventilate as they hibernate, the tissue CO2 stores increase, and intracellular pH becomes acidotic in most tissues. This acidotic state causes a further depression of metabolism that teleologically may be useful by further decreasing the energy consumption of nonfunctioning tissues, such as skeletal muscle, gastrointestinal tract, and higher brain centers. In contrast, active tissues, such as heart and liver, adopt a different strategy by actively extruding H+ across their cell membranes to maintain intracellular pH at or near the values predicted by the α-stat methodology. Therefore, hibernating mammals are able to vary their intracellular-to-extracellular pH gradient differently in different tissues, depending on the state of metabolic activity of the tissue. Functionally, this provides different types of acid-base regulation in different tissues, depending on the metabolic activity of the tissue. The first noticeable change associated with arousal from hibernation is hyperventilation. This depletes the CO2 stores, raises intracellular pH, and increases the metabolic rate. The animal reverts to an overall α-stat pH control pattern during awakening, which allows tissues to regain optimal function. Therefore, in hibernating mammals, the issue of acid-base maintenance is not clearly defined because intracellular acid-base regulation can be independent of blood regulation both within and among different tissues in the same animal.
Despite the preceding discussion, the practical question of how acid-base status should be regulated during hypothermic bypass in humans remains open. Some animal studies suggest that α-stat acid-base management is beneficial in terms of myocardial protection. McConnell et al. (24) evaluated α-stat regulation during hypothermia in dogs and demonstrated that significant elevations in coronary blood flow, left ventricular oxygen consumption, and lactate utilization occurred with maintenance of a pH of 7.7 at 28°C (α-stat) in comparison with a pH of 7.4 (pH-stat). There was also a significant increase in peak ventricular pressure when a standard preload was applied. Poole-Wilson and Langer (25) demonstrated a greater contractility in hypothermic perfused papillary muscle when the pH of the perfusate was more alkaline than 7.4. They also demonstrated a rapid fall in myocardial tension in addition to changes in Ca2+ flux when the perfusate PaCO2 was increased (26). On the other hand, Sinet et al. (27) found no effect of pH on the performance of isolated rat heart. The myocardium is often not perfused but is purposely made ischemic to facilitate cardiac surgery. In this setting, alkalinization of the blood before ischemia has been shown to decrease the development of acidosis in coronary sinus blood and improve contractility on reperfusion (28). It also appears that the pH of the blood reperfusing the heart may be critical to the recovery of ventricular performance. Becker et al. (29) studied the myocardial effects of an acid-base strategy in which alkalinization greater than that of α-stat was used. They found improvements in myocardial performance after 1 hour of circulatory arrest and cardioplegia, with moderate alkalinization in comparison with α-stat. Acid-base management also appears to be important in cardiac electrophysiology. Swain et al. (30) showed the electrical stability of the heart to be increased, with less spontaneous ventricular fibrillation, when α-stat blood regulation was compared with pH-stat. Kroncke et al. (31) found a 40% incidence of ventricular fibrillation in patients cooled to 24°C during pH-stat management and a 20% incidence in those managed with α-stat.
The appropriate acid-base management for optimal cerebral perfusion has also been questioned. Clearly, CBF decreases significantly with hypothermia. Cerebral metabolic rate also decreases during hypothermic bypass. The response of the cerebral circulation to changes in PaCO2 is preserved, at least during moderate hypothermia (32); therefore, α-stat management will result in lower cerebral flows than those seen with pH-stat management. However, because of the lowered metabolic demands, a lower CBF may be appropriate and indicative of a maintained coupling of blood flow and metabolic demand. Govier et al. (33) demonstrated intact autoregulation in humans using an α-stat strategy at temperatures ranging from 21°C to 29°C. Murkin et al. (34) showed coupling of CBF and metabolism that was independent of cerebral perfusion pressure (CPP) within the range of 20 to 100 mm Hg when α-stat management was employed. In contrast, cerebral autoregulation was abolished and CBF varied with perfusion pressure when pH-stat strategy was used (Fig. 8.7). It has been argued that the CBF during pH-stat hypothermia actually represents excessive blood flow and may be detrimental. Unnecessarily high blood flows may put the brain at risk of damage by microemboli or high intracranial pressure. With deep hypothermia (i.e., temperatures <20°C), the normal vascular responses are lost and CBF becomes pressure dependent (35,36). At deep hypothermic temperatures, coupling of cerebral flow and metabolism is also lost. It is important to note, however, that the responses of CBF and CMRO2 (cerebral metabolic rate of oxygen) are quantitatively different at deep hypothermic conditions. CBF decreases linearly with the decrease in temperature, whereas CMRO2 drops exponentially. The net result is that CBF becomes more luxuriant at deep hypothermic temperatures. At normothermia, the mean ratio of CBF to CMRO2 is 20:1, and at deep hypothermia, the ratio increases to 75:1 (37). This situation is important in the context of low-flow CPB. At very low temperatures, data indicate that pump flow rates may be reduced to as little as 10 mL/kg/min before flow becomes inadequate for cerebral metabolic requirements (38).
On a microcirculatory level, some evidence suggests that α-stat management may be beneficial to the brain. Norwood et al. (39) studied the brains of hypothermic dogs perfused with anoxic blood and found a decrease in extent and magnitude of lesions when the perfusate had a higher pH. Acidic perfusate enhanced the extent of the lesions. On the other hand, Priestley et al. (40) compared neurologic and histologic outcome in a survival piglet model of deep hypothermic circulatory arrest.
They showed that the pH-stat group demonstrated better neurologic performance and less severe functional disability scores than those piglets in the α-stat group. Histologic injury was also more severe in the α-stat group.
They showed that the pH-stat group demonstrated better neurologic performance and less severe functional disability scores than those piglets in the α-stat group. Histologic injury was also more severe in the α-stat group.
Theoretically, hypocarbia (and increased pH) result in a leftward shift of the oxyhemoglobin dissociation curve, which causes oxygen to be less readily available to the tissues. However, more oxygen is dissolved in the plasma during hypothermia, so that these two effects tend to cancel out each other. The relatively low CBF during α-stat management has still been shown to be in excess of cerebral metabolic needs (34).
In adults, the preponderance of evidence suggests either that CO2 management on CPB does not matter or that an α-stat strategy is advantageous. Bashein et al. (41) examined the influence of pH management in 86 adults in whom mild hypothermia was utilized (approximately 30°C). They found no difference in cardiac or neuropsychologic outcome regardless of acid-base management. It is important to realize, however, that it would have been unlikely for them to be able to demonstrate a difference in this study under the conditions of the study. The differences in PCO2 between the two groups amounted only to approximately 6 to 7 mm Hg, and the degree of hypothermia was not very profound. Contrast the scenario in their study to that of a patient in deep hypothermia, for whom the difference in PCO2 between the two strategies approaches 80 mm Hg! In addition, their analysis looked for differences in mean group performances rather than changes in individual patient performance, a methodology that may have decreased the sensitivity of the study to detect any existing difference. Three randomized prospective studies of moderate hypothermia in adults have demonstrated that postoperative neurologic or neuropsychological outcome is slightly, but consistently, better with α-stat management (42,43,44). The notion that α-stat management may be beneficial in this setting in adults makes sense if one considers that the most likely mechanism for neurologic injury in these patients is probably emboli. Therefore, α-stat management would be expected to provide lower CBFs that are more aligned with the cerebral metabolic rate and a lesser embolic load.
Although acid-base management is probably not as important when moderate hypothermic temperatures are used, it may be critical in the setting of deep hypothermia. Proponents of the α
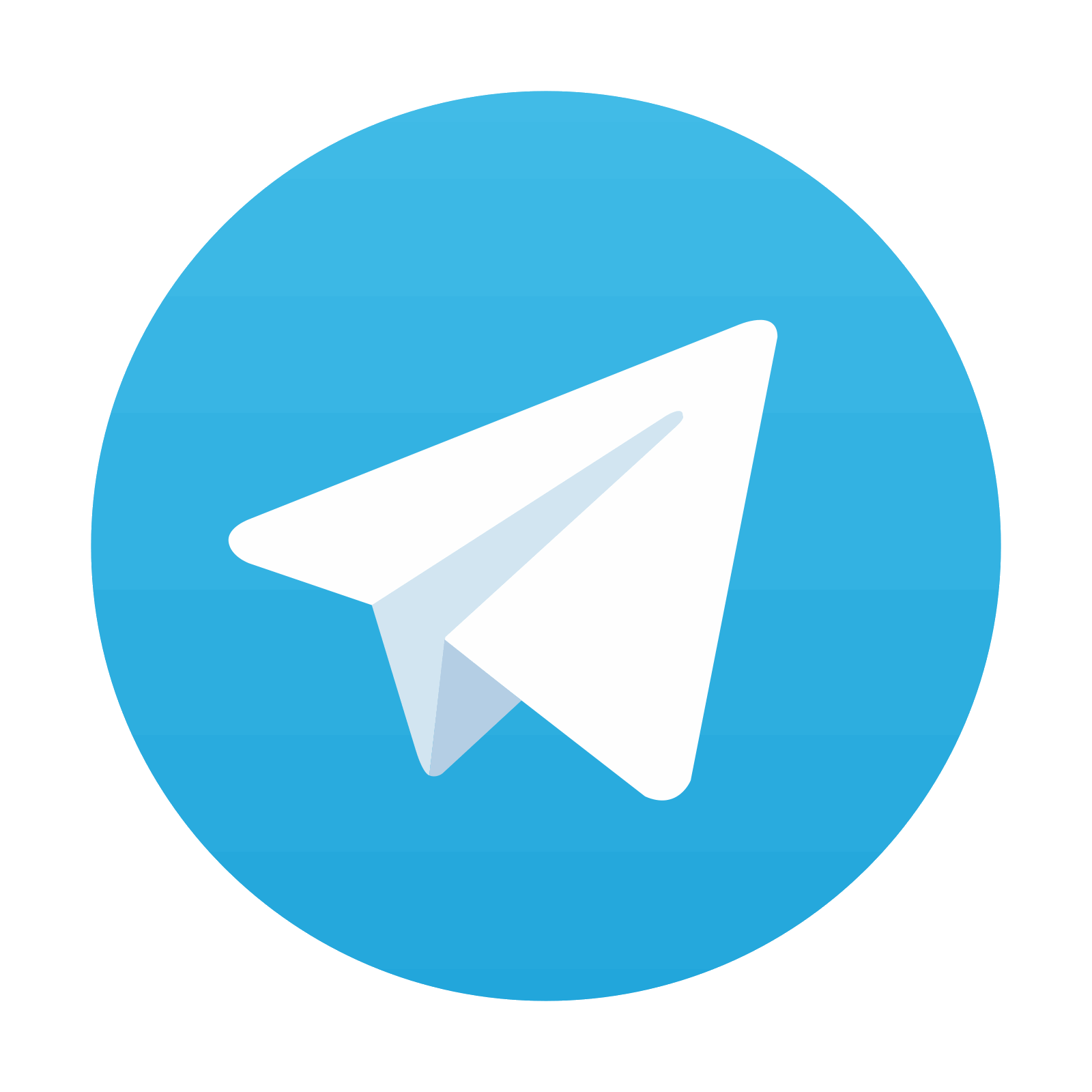
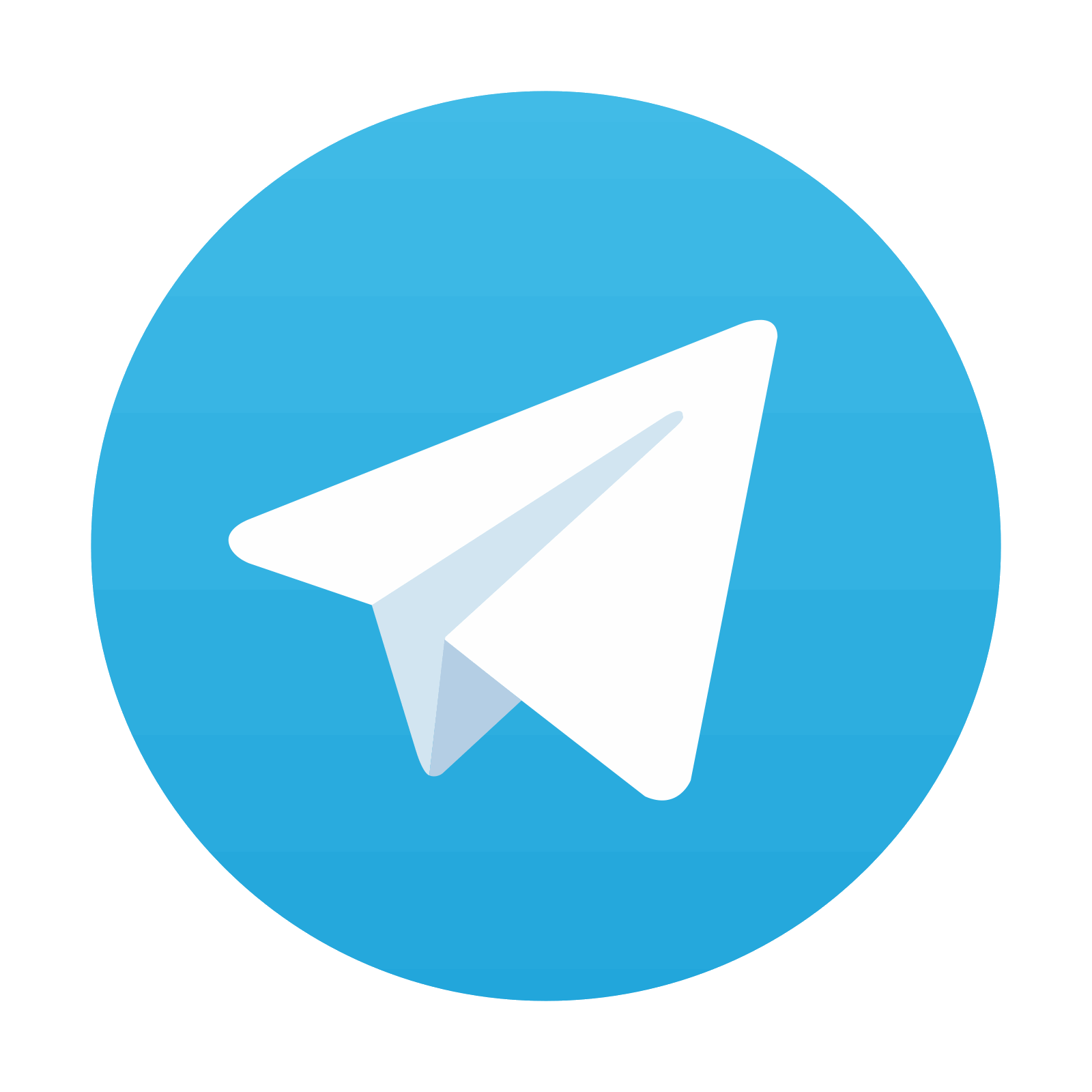
Stay updated, free articles. Join our Telegram channel
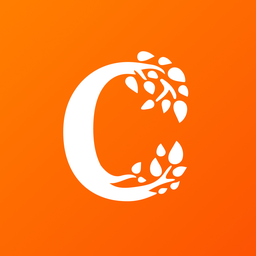
Full access? Get Clinical Tree
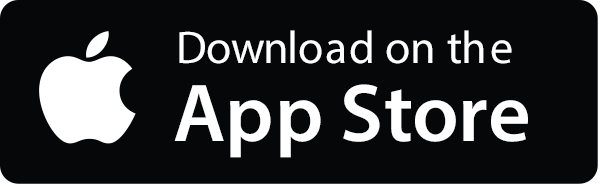
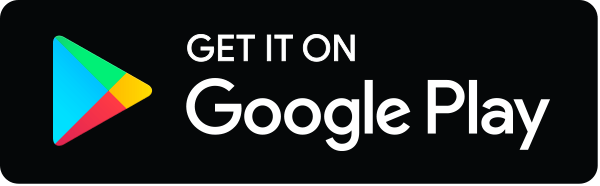
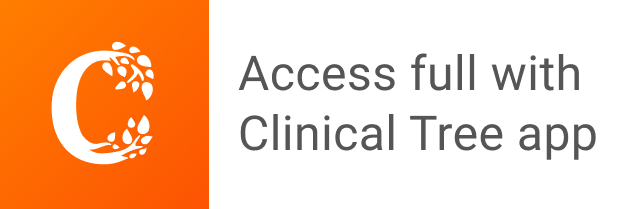