Introduction
The systemic circulation carries blood from the systemic ventricle through a network of arteries and arterioles to the tissue capillaries and drains it via the systemic venous system to the systemic venous atrium.
The systemic arterial system serves two important functions. First, it acts as a low-resistance conduit through which blood is distributed to different parts of body. Second, the arterial tree buffers the pulsatile pressure to convert the systemic ventricular pulsatile blood into a steady stream of capillary flow. Additionally, the endothelium, which lines the vascular lumen, exerts important vascular homeostatic effects through the production of a variety of substances. Hence alterations of the mechanical properties of the arterial wall and function of the endothelium have significant implications for normal functioning of the systemic arterial system and in the development of cardiovascular disease. Furthermore, optimal performance of the systemic ventricle depends on its favorable interaction with the systemic circulation. In the setting of congenital heart disease, the systemic ventricle may be a morphologic left ventricle, morphologic right ventricle, or single functional ventricular chamber of right, left, or indeterminate morphology.
This chapter discusses the systemic circulation from the structural, physiologic, and mechanical perspectives. Assessment of arterial function and structure and pediatric conditions associated with systemic arterial dysfunction are highlighted. Finally, the concept of ventriculoarterial interactions and their relevance in congenital and acquired heart disease in the young is described. The systemic venous system is discussed in Chapter 28 .
Systemic Arterial System
Normal Structure
The systemic arterial tree begins with the aorta, which ramifies into tributaries to perfuse all parts of body with the exception of hair, nails, epidermis, cartilages, and cornea. The large central arteries are protected within the thoracic and abdominal cavities, while peripheral conduit arteries run along the flexor surfaces in the upper and lower limbs, where they are less exposed to injury. The ascending aorta arises at the base of the left ventricle and gives off its first branches, the right and left coronary arteries. It continues as the aortic arch, from which the brachiocephalic, left common carotid, and left subclavian arteries arise. The thoracic descending aorta begins as a continuation of the aortic arch and penetrates the diaphragm to continue as the abdominal descending aorta. The celiac trunk and superior and inferior mesenteric arteries arise from the abdominal descending aorta to supply the liver and gastrointestinal tract, while the renal arteries branch off at right angles to perfuse the kidneys. The descending aorta bifurcates at its distal end into the right and left common iliac arteries, the latter bifurcating into the internal iliac artery to supply the pelvic organs and the external iliac artery, which continues as the femoral artery to supply the lower limbs. The aorta tapers from its origin to its termination at the iliac bifurcation, and branched daughter vessels are always narrower than the parent vessel, which has implications on wave reflection. The arterial ramifications end in arterioles, which then usually continue as capillaries. Beyond the major arterial branches, the total cross-sectional area increases progressively to the capillary bed. The proportion of cellular and structural components also varies along the arterial tree. Nevertheless, the arterial wall is made up of three constant layers: an internal tunica intima, a tunica media, and an external tunica adventitia.
The intima comprises the endothelium, a subendothelial layer, and an elastic membrane. The endothelium consists of a monolayer of cells that line the vascular lumen. Apart from forming a physical barrier between the circulating blood components and the vascular wall, the endothelial cells play a pivotal role in vascular homeostasis. The subendothelial layer is made up of fibroblasts and variable amount of collagen. The internal elastic membrane consists of a network of elastic fibers and forms a boundary with the media.
The media, usually the thickest layer of the arterial wall, is responsible for the mechanical properties of the vessel. Its structural components are vascular smooth muscle cells and extracellular matrix, the latter consisting of elastic lamellae, collagen fibers, structural glycoproteins, and ground substance. Vascular smooth muscle cells maintain vascular tone through contraction and relaxation, while the extracellular matrix of the media provides a structural framework for optimal functioning of the blood vessels.
The elastic fibers in the media, arranged in concentric lamellae that form the boundaries between layers of vascular smooth muscle cells, are 90% composed of elastin. Cross-linking of elastin confers elasticity to the arteries. In addition, elastin has been implicated in the control of proliferation and phenotype of smooth muscle cells. Elastin has an estimated half-life of more than 40 years in humans; its rate of synthesis is thought to be negligible in adulthood. Elastin, damaged by degenerative and pathologic processes, is unlikely to be replaced. Other constituents of elastic fibers include microfibrillar-associated glycoproteins and fibrillin. Fibrillin forms a microfibrillar network that serves as scaffolding for the deposition of elastin and assembly of elastic fibers. Fibulin-5, through its interactions with elastin and integrins, plays a critical role during elastic fiber development and is a potential therapeutic agent for the treatment of elastinopathies. Other structural glycoproteins in the arterial wall include fibronectin, vitronectin, laminin, entactin/nidogen, tenascin, and thrombospondin.
Collagens are composed of three polypeptide α chains arranged to form a triple helix, which confers tensile strength to the vessel wall. Types I and III collagen are the major fibrillar collagens in blood vessels, constituting about 90% of vascular collagens. Collagen is the stiffest component of the arterial wall, with an elastic modulus of 10 8 to 10 9 dyne/cm 2 . By contrast, the elastic modulus of elastin is in the order of 10 6 dyne/cm 2 . Hence the absolute and relative quantities of elastin and collagen contribute significantly to the stiffness of the arterial wall. Elasticity of the arterial wall is a nonlinear function of transmural pressure. Proposed models of this nonlinear function take into account the contribution of vascular smooth muscle cells, viscoelastic properties of the matrix proteins, residual stresses due to growth and remodeling, and gradual recruitment of collagen fibers with increasing pressure.
The ground substance is filled by proteoglycans. Proteoglycans are macromolecules that possess one or more linear glycosaminoglycan chains linked to a core protein. The proteoglycans in the vessel wall are hyaluronan, versican, biglycan, decorin, lumican, syndecans, fibroglycan, and glypican. The proteoglycans have diverse roles in the organization of connective tissue structure, regulating cellular activities and metabolism, permeability, filtration, and hydration, and controlling cytokine bioavailability and stability. Matrix metalloproteinases play a fundamental role in the degradation of vascular extracellular matrix during physiologic and pathologic vascular remodeling.
The distribution of structural components within the media varies along the arterial tree. With increasing distance from the heart, the elastin-to-collagen ratio falls and smooth muscle cells increase. Alterations of structural components of the media as a result of degeneration, genetic mutations, or imbalance between the synthesis and degradation of extracellular matrix have a significant impact on the mechanical properties of the vessels.
The adventitia contains mainly fibroblasts and collagen fibers and some elastic fibers. It contributes also to the elastic properties of arteries. Nutrient vessels, vasa vasorum, arise from a branch of the artery or from a neighboring vessel to ramify and distribute to the adventitial layer.
Endothelial Function
The endothelium comprises a monolayer of endothelial cells lining the vascular lumen. It is strategically located between circulating blood components and vascular smooth muscle cells to exert a pivotal role in vascular homeostasis. By producing a wide variety of substances, the endothelium regulates vascular tone, inhibits smooth muscle cell proliferation and migration, controls cellular adhesion, regulates inflammation, and exerts fibrinolytic and antithrombotic actions. The concept of endothelial function is also extended from the vascular lumen to the vascular wall and adventitia, which are supplied by vasa vasorum, considered to be an active intravascular microcirculation.
Nitric oxide, initially identified as the endothelium-derived relaxing factor, is the major vasodilating substance released by the endothelium. Nitric oxide is synthesized from L-arginine by the action of endothelial nitric oxide synthase, primarily in response to shear stress produced by blood flow. Cofactors including tetrahydrobiopterin and nicotinamide adenine dinucleotide phosphate are involved in nitric oxide production. Apart from shear stress, endothelial nitric oxide synthase is also activated by bradykinin, adenosine, vascular endothelial growth factor, and serotonin. Asymmetric dimethylarginine, on the other hand, is an endogenous inhibitor of nitric oxide synthase and may mediate the adverse effects of traditional risk factors on endothelial vasodilator function. Nitric oxide has a half-life of a few seconds in vivo. It diffuses from endothelial cells to exert its relaxation effects on vascular smooth muscle cells by activating guanylate cyclase, which in turn increases the production of cyclic guanosine monophosphate and leads to a reduction of the intracellular calcium concentration. Apart from regulating vascular tone through vasodilation, nitric oxide also mediates other important vascular homeostatic functions by exerting inhibitory effects on the proliferation of vascular smooth muscle, counteracting leukocyte adhesion to the endothelium, and inhibiting platelet aggregation.
The endothelium also mediates hyperpolarization of the vascular smooth muscle to cause relaxation. Although the identity of the endothelium-derived hyperpolarizing factor remains elusive, its hyperpolarizing mechanism is considered to be mediated by calcium-activated potassium channels on vascular smooth muscle. Candidates include epoxyeicosatrienoic acids, potassium ion, gap junctions, hydrogen peroxide, and C-type natriuretic peptide. It has been suggested that endothelium-derived hyperpolarizing factor might play a compensatory role for the loss of nitric oxide–mediated vasodilation in patients with heart failure. Other endothelium-derived vasodilators include prostacyclin and bradykinin. Prostacyclin is produced via the cyclooxygenase pathway and acts independently of nitric oxide to cause vasodilation. It also acts synergistically with nitric oxide to inhibit platelet aggregation. Prostacyclin appears to have a limited role in humans in the control of vascular tone. Bradykinin stimulates the release of nitric oxide, prostacyclin, and endothelium-derived hyperpolarizing factor.
Regulation of vascular tone by the endothelium is also accomplished by the control of vasoconstrictor tone through the release of endothelin and the conversion of angiotensin I to angiotensin II at its surface. Endothelin-1, the predominant endothelin isoform in the cardiovascular system, binds to ET A receptors on vascular smooth muscle cells to cause vasoconstriction. At lower concentrations, however, endothelin-1 causes transient vasodilation in the human forearm circulation, probably owing to the release of nitric oxide and prostacyclin via ET B receptors located on endothelial cells.
Vascular Smooth Muscle Function
Contraction of vascular smooth muscle cells reduces vessel diameter, increases vascular tone, and regulates blood flow by shortening the cells. This contractile phenotype is modified by the expression of genes that encode contractile proteins, ion channels, and other molecules involved in contraction. Smooth muscle contraction is regulated in vivo primarily by pharmacomechanical and electromechanical activation of the contractile proteins myosin and actin. Pharmacomechanical coupling refers to the activation of contraction by ligands of cell surface receptors without an obligatory change in the plasma membrane potential. The phosphoinositide signaling cascade is the common second-messenger system utilized by the surface receptors. Electromechanical coupling, on the other hand, involves alterations in the plasma membrane potential. Receptor activation may induce an activation of receptor-operated or voltage-dependent channels and lead to the passive influx of calcium down its concentration gradient. The balance between force generation and release is responsible for the maintenance of vascular tone. The vascular tone is influenced by local metabolic substances, humoral factors, and activity of the autonomic nervous system. A detailed discussion of the molecular mechanisms of smooth muscle contraction is beyond the scope of this chapter; however, interested readers are referred to recent published reviews.
Apart from a contractile phenotype, vascular smooth muscle cells exhibit other phenotypes. This phenotypic diversity plays an important role in the normal development, repair of vascular injury and in vascular disease process. After vascular injury, phenotypic modulation of vascular smooth muscle cells causes the upregulation of genes required for their proliferation and the production of extracellular matrix and suppression of genes that characterize the contractile phenotype. On the other hand, inappropriate pathologic differentiation into other mesenchymal lineages—such as osteoblastic, chondrocytic, and adipocytic ones—may contribute to vessel calcification, altered matrix production, and abnormal lipid accumulation, respectively. Studies have focused on the understanding of mechanisms that underlie the physiologic control and pathologic alterations of phenotypic switching of vascular smooth muscle cells.
Control of Circulation
The regulation of circulation aims to adjust the blood flow precisely to meet the needs of tissue and to maintain an adequate driving pressure to perfuse the various body tissues. Such control is achieved through local mechanisms, humoral factors, and neural regulation.
Local Control
Autoregulation refers to the ability to maintain a relatively constant blood flow in response to acute changes in perfusion pressure. The coronary, renal, and cerebral circulations exhibit autoregulation. Two theories have been proposed for this autoregulatory mechanism. The metabolic theory suggests that elevated perfusion pressure increases blood flow, and hence oxygen delivery and removal of vasodilators, thereby leading to vasoconstriction and reduction of blood flow and vice versa. The myogenic theory proposes that stretching of vascular smooth muscle cells by the elevated perfusion pressure increases their tension, which in turn causes vasoconstriction to reduce blood flow. Conversely, less stretching at lower perfusion pressure causes smooth muscle relaxation and increases blood flow. However, the exact mechanisms that link intraluminal pressure generation to myogenic constriction remain uncertain.
Metabolic mechanisms also contribute to the control of local blood flow. Two theories have likewise been proposed. The vasodilator theory proposes that vasodilator substances are formed and released from tissues when metabolic rate increases or oxygen and other nutrient supplies decrease. Possible vasodilator substances include adenosine, carbon dioxide, potassium ion, hydrogen ion, lactic acid, histamine, and adenosine phosphate. The nutrient theory suggests that blood vessels dilate naturally when oxygen or other nutrients are deficient. Hence increased utilization of oxygen and nutrients increases metabolism to cause local vasodilation, a phenomenon referred to as active hyperemia. Reactive hyperemia is another phenomenon related to the local metabolic flow-control mechanism. In reactive hyperemia, a brief interruption of arterial blood flow results in a transient increase in blood flow that exceeds the baseline, after which the flow returns to baseline level. Both the deprivation of tissue oxygen and accumulation of vasodilating substances probably account for this phenomenon. The duration of reactive hyperemia depends on the duration of flow cessation and usually lasts long enough to repay the oxygen debt.
Autoregulation and metabolic mechanisms control blood flow by dilation of the microvasculature. The consequent increase in blood flow dilates the larger arteries upstream via the mechanism of flow-mediated dilation. The pivotal role of endothelial cells in the transduction of shear stress secondary to increased blood flow and the release of the vasodilators has been alluded to earlier. Flow-mediated dilation occurs predominantly as a result of local endothelial release of nitric oxide. The mechanisms of shear stress detection and subsequent signal transduction are unclear but probably involve opening of calcium-activated potassium channels that hyperpolarizes endothelial cells and calcium activation of endothelial nitric oxide synthase. Flow-mediated dilation increases flow with a negligible increase in pressure gradient, thus optimizing energy losses within the circulation. The phenomenon of flow-mediated dilation as induced by reactive hyperemia has commonly been used as an assessment of endothelial function in vivo. All of the aforementioned mechanisms represent relatively acute responses to regulate local blood flow. Long-term local mechanisms involve changes in tissue vascularity, the release of angiogenic factors, and the development of collateral circulations.
Humoral Control
Humoral control refers to regulation by hormones or locally produced vasoactive substances that act in an autocrine or a paracrine fashion. These humoral substances act either directly via receptors on vascular smooth muscle cells or indirectly by stimulating the endothelium to release vasoactive substances.
Circulating catecholamines, noradrenaline and adrenaline, are secreted by the adrenal medulla, which is innervated by preganglionic sympathetic fibers. Sympathetic activation stimulates the release of catecholamines, about 80% being noradrenaline, from the adrenal gland. The adrenal gland and the noradrenergic sympathetic vasoconstrictor fibers provide dual control of the circulation by catecholamines. The adrenergic receptors in the blood vessels are α 1 , α 2 , and β 2 receptors. Noradrenaline causes vasoconstriction by acting on α-receptors, while adrenaline causes vasodilation at physiologic concentrations through its β-agonist effect. At higher concentrations, adrenaline also causes vasoconstriction by activating α-receptors.
The regulatory role of the renin-angiotensin system in the circulation is well known. The final effector of the system, angiotensin II, mediates its effects classically in an endocrine fashion. In response to decreased renal perfusion pressure or extracellular fluid volume, renin is secreted from the juxtaglomerular apparatus of the kidney and cleaves angiotensinogen, released from the liver, to form angiotensin I. By action of the angiotensin converting enzyme, which is predominantly expressed on the surface of endothelial cells in the pulmonary circulation, angiotensin I is converted to angiotensin II. Angiotensin II is a potent vasoconstrictor and acts directly by stimulating the angiotensin II type I (AT 1 ) receptor and indirectly by increasing sympathetic tone and the release of vasopressin. A local paracrine renin-angiotensin system also exists in the vasculature. Vascular production of angiotensin II has been shown to be mediated by the endothelium. The tissue renin-angiotensin system has dual effects on vessel function, being mediated through opposing effects of two receptors. Stimulation of AT 1 receptor causes contraction of vascular smooth muscle by directly increasing intracellular calcium and indirectly stimulating synthesis of endothelin-1 and other vasoconstrictors. Furthermore, promotion of oxidative stress via the AT 1 receptor may possibly reduce nitric oxide bioavailability. On the other hand, stimulation of angiotensin II type 2 receptor appears to mediate vasodilation by activating the nitric oxide pathway. The local tissue angiotensin II hence also plays an important role in maintaining vascular homeostasis. Other biologically active aminopeptides of the circulating renin-angiotensin system, such as angiotensins III and IV, may act in the central nervous system to raise blood pressure through the AT 1 receptor.
Three peptides of the natriuretic peptide family—atrial natriuretic peptide, brain natriuretic peptide, and C-type natriuretic peptide—also participate in the control of circulation. Atrial natriuretic peptide is primarily produced by the atrial myocardium, while brain natriuretic peptide is synthesized by the ventricular myocardium. The main stimulus for their release is stretching of the myocardium. Other stimuli include endogenous vasoactive factors, neurotransmitters, proinflammatory cytokines, and hormones. Atrial and brain natriuretic peptides reduce sympathetic tone through suppression of sympathetic outflow from the central nervous system, reduction of release of catecholamines from autonomic nerve endings, and probably damping of baroreceptors. The consequence is decrease in vascular tone and increase in venous capacitance. Both of these peptides also inhibit the activities of the renin-angiotensin system, endothelins, cytokines, and vasopressin. The renal hemodynamic effects include the induction of diuresis secondary to increased glomerular filtration due to vasodilation of afferent renal arterioles and vasoconstriction of the efferent arterioles and the promotion of natriuresis. Despite preload reduction, reflex tachycardia is suppressed as these peptides lower the activation threshold of vagal afferents. C-type natriuretic peptide is a more potent dilator of veins than the other natriuretic peptides and acts in an autocrine or paracrine fashion.
Adrenomedullin was first isolated from human pheochromocytoma cells. It is produced in a wide range of cells, including vascular endothelial and smooth muscle cells, and plays a significant role in the control of circulation. Infusion of adrenomedullin via the brachial artery in humans induces dose-dependent vasodilation to increase blood flow. Furthermore, blockade of the vasodilating effect of adrenomedullin by inhibition of nitric oxide synthase suggests that nitric oxide may be an important mediator for adrenomedullin.
The endothelium-derived vasoactive substances and their role in the control of vascular tone and homeostasis have already been discussed. The three classes of eicosanoids, prostaglandins, thromboxanes, and leukotrienes are generated by metabolism of arachidonic acid present in the phospholipids of cell membranes. Endothelial cells produce predominantly prostacyclin and lesser amounts of prostaglandin E 1 , also a vasodilator, and prostaglandin F 2α , a vasoconstrictor. Nonetheless prostacyclin appears to have a limited role in humans in the control of basal vascular tone. Thromboxane A 2 , although predominately generated by platelets, is also synthesized by the endothelium and induces vasoconstriction and platelet aggregation. Under normal physiologic conditions, eicosanoids—primarily prostacyclin, produced by the cyclooxygenase pathway—induce vasorelaxation. Furthermore, the cyclooxygenase-dependent vasodilators can compensate for the deficiency of other vasorelaxants. By way of the lipoxygenase pathway, leukotrienes are produced from arachidonic acid. Leukotrienes C 4 , D 4 , and E 4 cause arteriolar constriction, whereas leukotrienes B 4 and C 4 induce pulmonary vasoconstriction by activating cyclooxygenase to produce thromboxane A 2 .
Several other endogenous substances affect the systemic circulation. Vasopressin, produced in the supraoptic and paraventricular nuclei of the hypothalamus, is probably the most potent known endogenous constrictor. It is released in quantities sufficient to exert a pressor effect when volume depletion is significant but has little role in normal vascular control. Serotonin exists in large amounts in the enterochromaffin cells of the gastrointestinal tract. Although serotonin exerts vasoconstrictor and vasodilator effects, depending on the vasculature, its function in regulating the circulation is unknown. Kinins are among the most potent endogenous vasodilators; examples include bradykinins and kallidin. Bradykinin is believed to play a role in the control of blood flow in the skin, gastrointestinal glands, and salivary glands. Histamine is released from mast cells and basophils upon stimulation by injury, inflammation, or allergic reaction to induce vasodilation and increase capillary permeability.
Neural Control
Neural control of the systemic circulation involves feedback mechanisms that operate in both the short and long term through the autonomic, primarily the sympathetic, nervous system. Short-term changes in sympathetic activity are triggered either by reflex mechanisms involving peripheral receptors or by a centrally generated response. Long-term changes, on the other hand, are evoked through modulation of sympathetic nervous system by other humoral factors and possibly by central mechanisms involving the hypothalamus.
Peripheral receptors constitute the afferent limb of the reflex. These include arterial baroreceptors, arterial chemoreceptors, and cardiac stretch receptors. Arterial baroreceptors are located in the walls of the carotid sinus and aortic arch. Afferent fibers run in the glossopharyngeal and vagal nerves and terminate within the nucleus of the solitary tract. The neurons at the nucleus then excite neurons within the caudal and intermediate parts of the ventrolateral medulla to cause inhibition of the sympathoexcitatory neurons in the rostral ventrolateral medulla. Hence stretching of arterial baroreceptors increases afferent input and results in the reflex slowing of heart rate, a decrease in cardiac contractility, and vasodilation, thereby providing a negative feedback mechanism for the homeostasis of arterial pressure.
Peripheral chemoreceptors are located in the carotid and aortic bodies and are stimulated primarily by decreased arterial partial pressure of oxygen. Their afferent fibers also run in the glossopharyngeal and vagus nerves. Activation of peripheral chemoreceptor results in hyperventilation and sympathetically mediated vasoconstriction of vascular beds with the exception of those of the heart and brain. Hence oxygen conservation is attempted by increasing oxygen uptake and reducing tissue oxygen consumption. These chemoreflexes are subjected to negative feedback interaction, with inhibition of the chemoreflex-mediated sympathetic activation through the stimulation of baroceptors and thoracic afferents.
Atrial receptors are located in the walls of the right and left atria and in pulmonary venous and caval-atrial junctions. Two types of atrial receptors are described based on their discharge pattern in relation to atrial pressure changes. Type A receptors signal atrial contraction and hence respond to an increase in central venous pressure. These receptors send impulses via myelinated fibers in the vagus nerve, and the efferent portion consists of sympathetic activation. The tachycardia in relation to stimulation of sinuatrial node caused by atrial stretch is termed the Bainbridge reflex. Type B baroreceptors are stretch receptors stimulated by volume distension of the atria and their firing during ventricular systole. The afferents are unmyelinated vagal fibers. Atrial distension decreases sympathetic activity. Receptors that respond to stretch and contractility are also present in the ventricles. These receptors provide afferent input to the medulla via unmyelinated C fibers. Stimulation of these fibers decreases sympathetic tone and causes bradycardia and vasodilation. Stretching of the atrial and ventricular myocardium also leads to the release of natriuretic peptides, as discussed earlier.
Apart from the reflex-triggered short-term control of the circulation, the central pathways responsible for the central command responses—such as those occurring at the onset of exercise or evoked by a threatening stimulus—are now better understood. Evidence suggests the existence of a supramedullary integrative loop that connects the brain stem and paraventricular nucleus of the hypothalamus. The loop is composed of ascending noradrenergic projections from the nucleus of the solitary tract and caudal ventrolateral medulla and descending oxytocinergic and vasopressinergic neurons in the paraventricular nucleus of the hypothalamus projecting to brain stem areas. It is likely that reflex-triggered control interacts with the central command responses to regulate the cardiovascular response during exercise. Groups of neurons in the hypothalamus can project to synapse directly with sympathetic preganglionic fibers in the spinal cord, implying that the medullary vasomotor center is perhaps not the only region that directly controls sympathetic outflow.
The autonomic nervous system represents the efferent component of the neural control of the circulation. Up to three types of fibers may innervate blood vessels: sympathetic vasoconstrictor fibers, sympathetic vasodilator fibers, and parasympathetic vasodilator fibers. As the size of vessel decreases, the density of autonomic innervation increases. The small arteries and arterioles are therefore the most richly innervated arteries.
Sympathetic vasoconstrictor fibers release noradrenaline upon nerve stimulation and constitute the most important components in the neural control of the circulation. Postsynaptically the α 1 -adrenoceptor is the predominant receptor mediating vasoconstriction. Although noradrenaline is the principal neurotransmitter in the sympathetic nervous system, it coexists with adenosine triphosphate and neuropeptide Y in sympathetic neurons. Sympathetic vasoconstriction of arterioles increases vascular resistance, while constriction of capacitance vessels alters the circulating blood volume. In larger arteries, contraction of vascular smooth muscle in response to sympathetic activation causes less significant change in arterial caliber but alters vascular tone and hence arterial stiffness.
Sympathetic vasodilator fibers are scarce and not tonically active. Evidence suggests that sympathetic vasodilator fibers regulate skeletal vascular tone in many animal species. Both cholinergic and nitric oxide–dependent mechanisms contribute to the vasodilator effect. Parasympathetic vasodilator fibers are found in blood vessels of the salivary gland, cerebral arteries, and coronary arteries. The vasodilator effect is mediated via release of acetylcholine with hyperpolarization of the vascular smooth muscle.
Long-term neural regulation of the circulation is modulated by humoral and other factors. Angiotensin II is an important facilitator of sympathetic transmission. It may enhance neurotransmitter release at sympathetic nerve terminals, sympathetic transmission through sympathetic ganglia, and perhaps central activation of sympathetic nervous activity. Nitric oxide interacts with the autonomic nervous system at both the central and peripheral levels. Centrally, nitric oxide decreases sympathetic vasoconstrictor outflow. Peripherally, augmented vasoconstriction to nitric oxide synthase inhibition has been demonstrated in denervated forearm in humans. Interaction between nitric oxide and cholinergic vasodilator fibers is also evidenced by significant pressor response to nitric oxide synthase inhibition with cholinergic blockade. Finally, the hypothalamic paraventricular nucleus, which plays a role in the central command responses as discussed earlier, may mediate sustained increases in sympathetic nerve secondary to a variety of stimuli. Stress, anxiety, or pathologic conditions such as heart failure may hence exert a long-term influence on neural control of the circulation through the tonic activation of sympathoexcitatory neurons located in the paraventricular nuclei of the hypothalamus.
Modeling of the Systemic Circulation
Models
From the mechanical perspective, the systemic arterial system can be envisaged as a network of elastic tubes that receive pulsatile blood flow from left ventricular ejection and transmit it distally as a steady stream into capillaries. Hence, apart from acting as a low-resistance conduit, the systemic arterial tree functions as a cushion to smooth out pressure and flow pulsations generated by cycles of left ventricular contraction. Although the success of the conduit function depends primarily on a low peripheral vascular resistance, the efficiency of cushioning function depends on the elastic properties, described in terms of stiffness, of the arterial system.
Modeling of the arterial circulation has contributed significantly to the understanding of the behavior of the arterial system and the effects of arterial load on the systemic ventricle. The lumped model of arterial circulation, commonly termed the Windkessel model, was first described in the 18th century. In his book Haemastaticks , Hales drew an analogy between the arterial system and an air-filled dome of the fire engine compression chamber (Windkessel) ( Fig. 74.1 ). The cushioning function of the dome smooths out the pulsatile blood flow and protects the peripheral vascular beds from exposure to large fluctuations in pressure. The electrical analogues of the systemic arterial system are shown in Fig. 74.2 . The two-element electrical analogue of the Windkessel model comprises a capacitor, which represents the arterial compliance, and a resistor, the total peripheral resistance. The modified Windkessel model takes into account the input impedance (see later) of the proximal aorta by the addition of a resistor proximal the two-element capacitance-resistance model. A four-element Windkessel model, with the addition of an inertial term, has further been proposed and shown to be superior to the three-element Windkessel as a lumped model of the entire systemic tree. Inertance is due to the mass of the fluid and, physiologically, it can be regarded as the inertial effect secondary to simultaneous acceleration of the blood mass within the vessel. However, intrinsic shortcomings of the Windkessel models include the limitation of vessel elasticity to one site, lack of a finite velocity of propagation of the pulse wave, and failure to consider the significance of wave reflection.


The combination of the cushioning and conduit functions of the arterial tree results in two phenomena: (1) traveling of a pulse wave at a finite speed along the arterial wall and (2) wave reflection at arterial terminations and other discontinuities. A more realistic model of a distensible tube with one end receiving pulsatile ejection of blood from the left ventricle and with the other end representing the peripheral resistance has therefore been proposed. The pressure wave at any point along the tube represents the result of the incident and reflected waves. Elasticity of the tube determines the velocity at which the pulse travels and the timing of arrival of the reflected wave. When the tube is distensible, the wave velocity is slow and the reflected wave returns late in diastole. With stiffening of the tube, the pulse velocity increases and the reflected wave arrives earlier to merge with the systolic part of the incident wave and results in a higher systolic pressure and a lower diastolic pressure. Vascular stiffness is therefore an important mechanical property of the arterial tree and contributes to left ventricular afterload.
Arterial Impedance as Ventricular Afterload
Ventricular afterload can be conceptualized as all the external factors that oppose ventricular ejection and contribute to myocardial wall stress during systole. The hydraulic load of the systemic arterial system has therefore been taken to represent the afterload presented to the systemic ventricle. The total arterial hydraulic load comprises three components: resistance, stiffness, and wave reflection, all of which can be obtained from impedance spectra based on analysis in the frequency domain.
Vascular Resistance
Vascular resistance is commonly used in the clinical setting as an index of systemic ventricular afterload. The electrical analogue for vascular resistance is described by the Ohm’s law, which applies to direct electric current circuit. For a steady flow state, the vascular resistance is derived by dividing pressure gradient by volume flow. As the systemic venous pressure is very small when compared with the mean aortic pressure, the systemic arterial resistance can be approximated as mean aortic pressure divided by cardiac output. Nonetheless, as arterial blood flow is pulsatile in nature, the use of vascular resistance alone to describe afterload is deemed inadequate.
Vascular Impedance
For pulsatile flow, the corresponding pressure-flow relationship is vascular impedance. This is analogous to the voltage-current relationship of an alternating current electrical circuit. To analyze the mathematical relationship between pressure and flow waves, Fourier analysis is used to decompose these complex nonsinusoidal waves into a set of sinusoidal waves with harmonic frequencies that are integral multiples of the fundamental wave frequency.
Vascular input impedance is defined as the ratio of pulsatile pressure to pulsatile flow. The aortic input impedance is particularly relevant as it characterizes the mechanical property of the entire systemic arterial circulation and represents the hydraulic load presented by the systemic circulation to the left ventricle. To obtain the aortic input impedance spectrum, the ascending aortic flow is measured by an electromagnetic flow catheter, while the pressure is measured by a micromanometer mounted onto the catheter. Noninvasive determination of aortic input impedance involves the use of Doppler echocardiography to measure flow and tonometry to obtain a carotid, subclavian, or synthesized aortic pressure waveform, the latter based on the radial arterial waveform. An example of the human aortic input impedance spectra is shown in Fig. 74.3 . For a heart rate of 60 beats/min, the fundamental frequency is 1 Hz, the second harmonics is 2 Hz, and so forth. The vascular impedance modulus at different harmonics is the ratio of pressure amplitude to flow amplitude. The phase difference is the delay in phase angle between the pressure and flow harmonics, which is analogous to time delay in the time domain.

The impedance at zero frequency is equivalent to resistance in the steady-flow state. Characteristic impedance is the ratio of pulsatile pressure to pulsatile flow at a site where pressure and flow waves are not influenced by wave reflection. The concept of characteristic impedance is important as it is related directly to stiffness of the major arteries distal to the site of measurement. Hence it represents the pulsatile component of the hydraulic workload presented to the left ventricle when measured at the ascending aorta. As wave reflection is always present, characteristic impedance cannot be measured directly. It is usually estimated by averaging impedance moduli over a frequency range where fluctuations due to wave reflection above characteristic impedance are expected to cancel out those below. Hence characteristic impedance has been estimated as the average value of moduli between 2 and 12 Hz, above 2 Hz, or above the frequency of the first minimum.
Wave Reflection
As the velocities of pressure and flow waves transmitted in the arteries are in the order of meters per second, it is obvious that the waves have sufficient time to travel to the periphery and be reflected back before the next cardiac cycle. The terminations at where low-resistance conduit arteries terminate in high-resistance arterioles are usually regarded as the principal sites for reflection. Possible reflecting sites include branching points in major arteries, areas of alterations in arterial stiffness, and high-resistance arterioles.
The pressure and flow waves measured at any site in the arterial system can be envisaged as a summation of a forward or incident wave and a reflected wave. Wave reflection exerts opposite effects on pressure and flow. Reflected pressure wave increases the amplitude of the incident pressure wave, whereas a reflected flow wave decreases the amplitude of the incident flow wave. In most experimental animals and in young human subjects who have elastic arteries, wave reflection returns to the ascending aorta from the periphery after ventricular ejection. Such timing is desirable, as the reflected pressure wave augments early diastolic blood pressure and contributes to aortic valve closure, thereby boosting the perfusion pressure of the coronary arteries without increasing left ventricular afterload. Stiffening of the systemic arteries due to aging or disease processes, however, increases pulse-wave velocity and causes an earlier return of the reflected wave to augment aortic blood pressure in late systole rather than in diastole. The implications of this pressure augmentation are discussed in the section on ventriculoarterial interaction further on.
Measurement of Arterial Function
Arterial Stiffness
Arterial stiffness describes the rigidity of the arterial wall. It is primarily determined by the structural components of the arterial wall, elastin and collagen in particular, vascular smooth muscle tone, and transmural distending pressure. The endothelium also plays a role in the regulation of arterial stiffness through the release of vasoactive substances to alter the smooth muscle tone. The significance of arterial stiffness stems from its direct relationship to characteristic impedance, hence the pulsatile component of the arterial afterload, and its effect on the timing of return of the reflected waves from peripheral sites.
In adults, the role of arterial stiffening in the development of cardiovascular disease is recognized. Associations between increased arterial stiffness and various pathophysiologic conditions, which are themselves also associated with increased cardiovascular risk, in adults has been extensively reviewed. Importantly, stiffness of central arteries, as assessed by aortic pulse-wave velocity and carotid distensibility, has been shown to have independent predictive value for cardiovascular events in the general adult population, in the elderly and adults with hypertension, end-stage renal disease, and impaired glucose tolerance.
Although stiffness of the central arteries has been the focus of adult studies, the contribution of stiffness of the smaller peripheral arteries to total vascular impedance cannot be ignored. Structural remodeling occurs also in smaller arteries and branching points, and changes in the mechanical properties of conduit and resistive arteries influence wave reflections and contribute to augmentation of late systolic blood pressure in the aortic root. Associations between increased small artery stiffness, as assessed by pulse contour analysis, and aging, hypertension, smoking, diabetes, and cardiovascular events have also been reported. Indeed, the mapping of arterial stiffness at multiple sites may provide a holistic approach to the prediction of cardiovascular events.
The increasing application of noninvasive methods to determine systemic arterial stiffness in the clinical and research arenas has significantly increased the understanding of its pathophysiologic significance. With adoption of these noninvasive methodologies for use in children and adolescents, the significance of arterial stiffening in the young is also being increasingly understood.
Measurement of Arterial Stiffness in vivo
Noninvasive methods for the determination of local, regional, and systemic arterial stiffness and the quantification of wave reflections in vivo are available. For meaningful interpretation of these indexes, their fundamental limitations have to be taken into account. First, the relationship between pressure and arterial diameter is nonlinear due to progressive recruitment of the stiffer collagen as transmural pressure increases. Second, modulation of smooth muscle tone by sympathetic nervous activity, hormones, or endothelium-derived vasoactive substances as previously mentioned can alter arterial stiffness. Finally, spontaneous vasomotor changes in the muscular arteries can alter arterial diameter and stiffness.
Local Arterial Stiffness
Local arterial stiffness is obtained by relating pressure changes to arterial diameter or cross-sectional area changes at the site of interest. Arterial stiffness can be expressed as compliance, distensibility, Peterson elastic modulus, Young’s modulus, and stiffness index ( Table 74.1 ). Among the various indexes of local arterial stiffness, the stiffness index is considered relatively independent of systemic blood pressure.
Term | Definition | FORMULA | |
---|---|---|---|
Change in Vessel Diameter | Change in Cross-Sectional Area of Vessel Lumen | ||
Compliance | Absolute change in diameter or area during systole for a given pressure change | ΔD/ΔP | ΔA/ΔP |
Distensibility | Relative change in diameter or area during systole for a given pressure change | ΔD/(D • ΔP) | ΔA/(A • ΔP) |
Peterson elastic modulus | Inverse of distensibility (i.e., the pressure change required for a given relative change in diameter or area) | ΔP • D/ΔD | ΔP • A/ΔA |
Stiffness index (β) | Ratio of ln (systolic/diastolic pressure) to relative change to vessel diameter | ln (Ps/Pd)/(ΔD/D) | |
Young’s modulus or incremental elastic modulus | Elastic modulus per unit wall thickness or area; provides information on intrinsic elastic properties of the arterial wall | ΔP • D/ΔD • h | [3(1 + A/WCSA)]/cross-sectional distensibility |
For superficial arteries such as the brachial, femoral, and carotid arteries, the diameter and its change from end-diastole to systole can be assessed by ultrasound and echo-tracking techniques. Two-dimensional ultrasound assessment is limited by the precision of measurements. By contrast, echo-tracking devices process radiofrequency signals to track the displacement of the anterior and posterior arterial walls with high precision ( Fig. 74.4 ). The precision in determining the change in diameter has been estimated to be as small as 1 µm for echo-tracking devices and about 150 µm for video-image analysis of ultrasound images. For deeper arteries such as the aorta, cine magnetic resonance imaging and transesophageal echocardiography have been used to determine diameter change during the cardiac cycle.

Ideally the local pressure should be measured at the site of diameter measurements. Applanation tonometry allows noninvasive recording of the arterial pressure waveform in the carotid and peripheral conduit arteries. The recorded pressure waveform can be calibrated against the cuff mean and diastolic blood pressures of the brachial artery. Derivation of the central aortic waveform from radial arterial tonometry has been made possible by the application of a transfer function, which has been validated in adults but not in children. Although the cuff brachial artery pulse pressure is commonly used for the calculation of local arterial stiffness indexes, amplification of pressure pulse along the arterial tree constitutes a potential source of error.
Several groups have reported the use of oscillometric blood pressure measurements for the noninvasive assessment of local arterial stiffness. The magnitude of the oscillation as a result of the blood vessel pulsation reflects the volumetric change of the underlying blood vessel. A stiffness parameter can be derived from the change in the cuff pressure in relation to the change in volume as represented by the accumulated magnitude of oscillation at each of the cuff pressure.
Recently tracking of vessel wall motion by ultrasound technologies has been used to interrogate vascular mechanics. Two-dimensional speckle tracking of arterial wall motion enables the quantification of global circumferential strain and strain rate and time to peak strain ( Fig. 74.5 ). A stiffness index that relates global circumferential strain to blood pressure can be calculated by the formula: ln (systolic blood pressure/diastolic blood pressure)/circumferential strain. Integration of the tissue Doppler-derived velocity data of the anterior and posterior arterial walls also allows the derivation of regional arterial strain and strain rate.

Regional Arterial Stiffness
Stiffness of an arterial segment, or regional stiffness, is assessed by measuring the pulse-wave velocity over the segment of interest. Pulse-wave velocity is the speed at which the pressure or flow wave is transmitted along the arterial segment. It is related to Young’s elastic modulus (E) by the Moens-Korteweg equation:
PWV=Eh/2rρ
where PWV is pulse-wave velocity, h is wall thickness of the vessel, r is the inside radius of vessel, and ρ is the density of blood. The Bramwell and Hill equation relates pulse-wave velocity to arterial distensibility:
PWV=(ΔP·V)/ΔVρ=1/ρD
where P is pressure, V is volume, Δ P • V /Δ V represents volume elasticity, and D is volume distensibility of the arterial segment. Furthermore, pulse-wave velocity is directly related to characteristic impedance ( Z c ) by the formula : Z c = PWV • ρ. Pulse-wave velocity is hence related directly to arterial elasticity and characteristic impedance and inversely to arterial distensibility. By providing an average stiffness of the arterial segment of interest, pulse-wave velocity may provide a better reflection of general vascular health.
Pulse-wave velocity is determined by dividing the distance of pulse travel by the transit time. The arterial pulse may be registered using pressure-sensitive transducers, an oscillometric device, applanation tonometry, Doppler ultrasound, or photoplethysmography. Furthermore, the pulse wave can be detected using magnetic resonance imaging, which also allows accurate determination of path length and measurements to be made from relatively inaccessible arteries.
Transit time is measured as the time delay between the feet of the proximal and distal pulse waves ( Fig. 74.6 ). The foot of the pulse wave is used to locate the wavefront as it is relatively unaffected by wave reflections. The most consistent method for determination of the foot of the pulse wave has been shown to be either the point at which its second derivative is maximum or the point formed by intersection of a line tangential to the initial systolic upstroke of the waveform and a horizontal line through the minimum point.

The distance is usually estimated by direct superficial measurement between the centers of the two pressure transducers or other devices. The method of measuring distance, however, varies. Some investigators use the total distance between the carotid and femoral sites of measurement, while others subtract the carotid-sternal notch distance from the total distance or subtract the carotid-sternal notch distance from the femoral-sternal notch distance. A recent expert consensus document suggests using 80% of the directly measured carotid-femoral distance in adults.
Although the carotid-femoral pulse-wave velocity is regarded as the gold standard measurement of arterial stiffness, pulse-wave velocities of the carotid-brachial, brachial-radial, and femoral–dorsalis pedis segments have also been measured. Simultaneous placement of oscillometric pressure cuffs at the brachia and ankles is also used to derive the brachial-ankle pulse-wave velocity that takes into account of both central and peripheral arterial stiffness. Despite the limitation of the need to estimate distance by superficial measurement, pulse-wave velocity is probably the most widely used technique for the assessment of arterial stiffness.
Systemic Arterial Stiffness
Pulse contour analysis is used to assess systemic or whole-body arterial stiffness noninvasively. One of the methods, based on the electrical analogue of a modified Windkessel model, concentrates on analysis of the diastolic pressure decay of the radial pulse contour. Based on the diastolic portion of the pulse contour, the capacitative compliance of the proximal major arteries and the oscillatory compliance of the distal small arteries are estimated. These parameters have been shown to change with aging and in diseases associated with increased cardiovascular events. The area method, based on a two-element Windkessel model, has also been used to determine systemic arterial compliance using the formula compliance = Ad/[TVR × (Pes − Pd)], where Ad is area under the diastolic portion of the central arterial pressure waveform from end-systole to end-diastole, TVR is total vascular resistance, Pes is end-systolic pressure, and Pd is end-diastolic pressure. The biologic relevance of these parameters of arterial stiffness is, however, unclear.
Wave Reflection Indexes
Arterial stiffening increases pulse-wave velocity and shortens the time for the pulse wave to return from the periphery. Early arrival of the reflected waves augments systolic blood pressure in stiff arteries. The effects of wave reflection can be quantified by determination of the augmentation index, which is defined as the ratio of difference between systolic peak and inflection point to pulse pressure ( Fig. 74.7 ). The inflection point corresponds to the time when peak blood flow occurs in the artery. In adolescents and young adults with elastic arteries, the augmentation index is negative as late return of reflected waves during diastole causes the peak systolic pressure to precede an inflection point. By contrast, in middle-aged and older individuals, the peak systolic pressure occurs in late systole after an inflection point, and the augmentation index becomes increasingly positive with age. Apart from arterial stiffness, the amplitude of the reflected wave, reflectance point, heart rate, and ventricular contractility are all important determinants of augmentation index. As height is related to reflection sites, the augmentation index is inversely related to height.

The aortic augmentation index can be determined noninvasively from the central aortic waveform. The latter is commonly estimated from the common carotid artery waveform obtained from applanation tonometry or sensors with multiple micro-piezoresistive transducers. Alternatively, the aortic waveform can be reconstructed using a transfer function from the radial waveform. The radial-to-aortic transfer function has nonetheless not been validated in children and, furthermore, its accuracy for derivation of aortic augmentation index has been debated.
Contour analysis of the digital volume pulse waveform has also been used to derive wave reflection indexes. The first peak in the waveform is thought to correspond to a forward-traveling pressure wave from the heart to the finger, and the second peak or point of inflection to the backward-traveling reflected pressure ( Fig. 74.8 ). A reflection index, defined at the ratio of the magnitude of the reflected wave to the first peak, has been proposed as a measure of the amount of reflection, while the peak-to-peak time has been used as a surrogate measure of pulse-wave velocity and arterial stiffness. The second derivative of the digital photoplethysmogram waveform has also been used to generate five distinct waves, the ratios of which have been related to age, augmentation index, and arterial stiffness. However, the physical and physiologic meanings of these measurements remain unclear.

Blood Pressure Indexes
Central pulse pressure is often considered a surrogate of arterial stiffness. It should not, however, be used interchangeably as an index of arterial stiffness, as their physiologic meanings differ. Similar to the augmentation index, central pulse pressure is dependent on heart rate, ventricular contractility, and factors affecting the reflected wave, notably arterial stiffness and reflectance points.
An ambulatory index of arterial stiffness has been derived from ambulatory blood pressure monitoring data gathered throughout the day and calculated as 1 minus the regression slope of diastolic blood pressure on systolic blood pressure. This is based on the concept that average distending pressure varies during the day and that the relation between diastolic and systolic blood pressure depends largely on the structural and functional characteristics of the large arteries. A recent systematic review and meta-analysis demonstrate its association with pulse-wave velocity and its ability to predict future cardiovascular events. Nevertheless the physiologic meanings of this index and its use as a marker of stiffness remain highly debatable.
Endothelial Dysfunction
Endothelial dysfunction is characterized by upset of the regulation of balance between vasodilation and vasoconstriction, inhibition and promotion of vascular smooth muscle proliferation, and prevention and stimulation of platelet aggregation, thrombogenesis, and fibrinolysis by the endothelium. Although the normal quiescent state is represented by the nitric oxide–dominated endothelial phenotype and maintained primarily by laminar shear stress, endothelial activation is characterized by dominance of reactive oxygen signaling. The common denominator of chronic production of reactive oxygen species exhausts the protective capacity of endogenous antiinflammatory and antioxidative mechanisms and results in sustained endothelial dysfunction. Dysfunction of the endothelium results in loss of its protective function, increased expression of adhesion molecules, and promotion of inflammation within the vessel wall.
Given the important protective role of the endothelium against vascular injury, inflammation, and thrombosis, all of which are key events involved in the initiation and progression of atherosclerosis, it is not surprising that endothelial dysfunction has prognostic implications. In adults with or without coronary atherosclerosis and in those with hypertension, the following have been shown to predict cardiovascular events: coronary endothelial dysfunction, impaired flow-mediated dilation of the brachial artery, and impaired agonist-mediated increase in forearm blood flow. With the introduction of a variety of noninvasive techniques, as elaborated further on for assessment of endothelial function, the phenomenon of endothelial dysfunction has also been documented in an increasing number of pediatric and adolescent conditions.
Assessment of Endothelial Function in vivo
Coronary Circulation
Assessment of endothelial function in the coronary circulation was first described in 1986 by Ludmer et al., who demonstrated that local infusion of acetylcholine dilates angiographically normal epicardial coronary arteries secondary to release of nitric oxide from an intact endothelium. By contrast, acetylcholine was found to cause paradoxical constriction of atherosclerotic coronary arteries as a result of direct muscarinic action on vascular smooth muscle. Endothelial function of the coronary resistance vessels can be assessed simultaneously using Doppler flow wires. The measurement of changes in coronary arterial diameter, blood flow, and vascular resistance in response to the intracoronary infusion of acetylcholine has become the gold standard against which other tests of endothelial function have been compared. Endothelium-independent changes in coronary diameter and flow reserve can be assessed by intracoronary boluses of adenosine or infusion of nitroglycerine. The response to endothelium-independent agonists is assessed to exclude insensitivity of vascular smooth muscle to nitric oxide. In children, coronary endothelial function has been mainly assessed in those with a history of Kawasaki disease (see later). The invasive nature of this technique, however, limits its use to patients in whom cardiac catheterization is clinically indicated and precludes serial follow-up assessments.
Forearm Resistance Vessels
Endothelial function of forearm resistance vessels is assessed by measuring forearm blood flow in response to the intra-arterial infusion of endothelium-dependent and -independent agonists. Venous occlusion plethysmography has been widely used to measure forearm blood flow. This is based on the premise that interruption of venous outflow from the forearm, but not arterial inflow, results in a linear increase in forearm volume, which can be measured using strain-gauge plethysmography.
A variety of agonists—including acetylcholine, methacholine, bradykinin, 5-hydroxytryptamine, and substance P—have been used to assess the endothelial-dependent vasodilation response. Apart from the stimulation of nitric oxide release, these agonists also induce the release of endothelium-derived hyperpolarizing factor and prostaglandins. The response to endothelium-independent agonist, like sodium nitroprusside, is assessed to exclude abnormal vascular smooth muscle function. The forearm blood flow response to endothelium-dependent agonist has been found to correlate with coronary endothelial function and to independently predict cardiovascular events in patients with coronary artery disease. Basal release of nitric oxide in the basal forearm can also be assessed using this technique by infusing NG-monomethyl- l -arginine, an l -arginine analogue that inhibits nitric oxide synthase. Coinfusion of NG-monomethyl- l -arginine with specific endothelium-dependent agonist can further be used to determine upregulation of alternative vasodilator pathways in condition of endothelial dysfunction. Although invaluable in elucidating mechanisms that underlie endothelial dysfunction, this technique has limited applicability in children owing to the need for arterial cannulation.
Conduit Artery
Noninvasive assessment of flow-mediated dilation of the brachial artery using high-resolution ultrasound was first introduced by Celermajer et al. in 1992, based on the principle of endothelium-dependent release of nitric oxide in response to shear stress. In this technique, the brachial artery diameter and Doppler-derived flow velocity are determined at baseline when the subject has rested in a supine position for at least 10 minutes and after an increase in shear stress induced by reactive hyperemia.
To induce reactive hyperemia, a sphygmomanometer cuff, placed either above the antecubital fossa or over the forearm, is inflated to suprasystolic blood pressure and deflated after 4 to 5 minutes. Cuff occlusion of the upper arm has an additional direct ischemic effect on the brachial artery. There is no consensus, however, as to whether cuff occlusion of the upper arm or forearm provides more accurate information. Reactive hyperemia after cuff deflation increases shear stress and leads to dilation of the brachial artery. The maximum increase in flow is assessed within the first 10 to 15 seconds after cuff release, whereas the brachial artery diameter is usually measured at 60 seconds after cuff deflation, at a time when maximal dilator response occurs in normal subjects ( Fig. 74.9 ). Flow-mediated dilation in the radial, femoral, and posterior tibial arteries can similarly be determined by inflating the cuff at the wrist, just beneath the popliteal fossa, and the ankle, respectively. At least 10 minutes after cuff release, sublingual nitroglycerine is given to assess endothelium-independent vasodilation. This direct vasodilation response peaks at 3 to 5 minutes after the administration of nitroglycerine.

The methodologic issues, strengths, and limitations of this technique have been explored in depth by the International Brachial Artery Reactivity Task Force and the Working Group on Endothelin and Endothelial Factors of the European Society of Hypertension. The noninvasive nature of the technique and its correlation with coronary endothelial function have led to its widespread use in clinical trials and in the field of vascular epidemiology. This technique is also widely used for the assessment of endothelial function in children and adolescents, although its application in children below 6 years of age is likely to be difficult given the cooperation needed for accurate measurement of brachial artery diameter and flow.
The aforementioned techniques primarily assess the endothelial-dependent nitric oxide–mediated vasodilation response to agonists. Other techniques attempted to track the change in arterial stiffness upon endothelial stimulation. Salbutamol, a β2-agonist, has been demonstrated to reduce arterial stiffness in a nitric oxide–dependent manner. Thus the changes in augmentation index and the inflection point in the photoplethysmographic digital volume pulse in response to salbutamol inhalation have been used as measures of endothelial function. These techniques have been found to be much less reproducible in children as compared with brachial artery flow-mediated dilation.
Recently there is increasing interest in the assessment of endothelial function at rest by determination of low-flow–mediated constriction. This involves the assessment of changes in brachial artery diameter in response to reduction of blood flow and shear stress after cuff occlusion of the distal artery. Associations between this parameter and traditional cardiovascular risk factors and severity of coronary artery disease have been demonstrated. Low-flow–mediated constriction has been proposed to result from reduced release of prostaglandins and endothelium-derived hyperpolarizing factor and increased production of endothelin-1.
Microvasculature
Laser Doppler techniques are used to assess the microvascular endothelial function of the skin. These techniques determine the magnitude of increase in red blood cell flux in the skin during reactive hyperemia after brief arterial occlusion, during local thermal hyperemia, and after local application of endothelium-dependent vasodilators by iontophoresis. Hence acetylcholine iontophoresis coupled with laser Doppler imaging have been used to assess microvascular endothelial function in neonates.
Reactive hyperemia peripheral arterial tonometry, which determines the change in digital pulse-volume amplitude during reactive hyperemia, is also used to assess peripheral microvascular endothelial function ( Fig. 74.10 ). The role for nitric oxide in the augmentation of pulse-volume amplitude during reactive hyperemia in humans has been demonstrated. Large cross-sectional studies in adults have shown that digital vascular dysfunction is associated with cardiovascular risk factors, though having little or no association with brachial artery flow-mediated dilation. The feasibility and reproducibility of peripheral arterial tonometry have been demonstrated in adolescents, although data on its usefulness in the pediatric population remain limited.

The magnitude of reactive hyperemia in response to induced shear stress on the endothelium of conduit arteries is also regarded as a measure of peripheral microvascular function. This is commonly quantified by the velocity-time integral of hyperemic flow adjusted for heart rate, which has been shown to be associated with cardiovascular risk factors and outcomes.
Circulating Biomarkers
The circulating pool of nitric oxide as a measure of its bioavailability can be assessed by measuring plasma nitroso compounds and nitrite. Although the exact chemical species of this pool are unclear and their values may be confounded by diet, studies have shown that plasma nitrite reflects regional endothelial nitric oxide synthase activity. Plasma nitrite reserve during reactive hyperemia and plasma nitroso compounds have been found to be reduced in the presence of endothelial dysfunction. Reduced nitric oxide availability can also be inferred from elevated levels of dimethylarginine, the naturally occurring antagonist of nitric oxide synthase, which has been shown to be associated with cardiovascular disease and mortality in adults. Activation of endothelial cells upregulates the expression of adhesion molecules, resulting in increased circulating levels of intercellular adhesion molecule 1, vascular cell adhesion molecule 1, E-selectin, and P-selectin. The levels of inflammatory markers—including high-sensitivity C-reactive protein, interleukin-6, tumor necrosis factor-α, and markers of oxidative stress, such as oxidized low-density lipoprotein and 8-iso-prostaglandin F2α—can be used to reflect ongoing endothelial activation and vascular inflammation.
Disturbance of the protective role of the endothelium against thrombosis in endothelial dysfunction is reflected by the imbalance between endothelium-derived tissue plasminogen activator and plasminogen activation inhibitor-1 and release into circulation of von Willebrand factor. Measurement of the dynamic release of tissue-plasminogen activator by agonists such as bradykinin, substance P, methacholine, and desmopressin has been used to assess endothelial function, although data on its relationship with cardiovascular risk factors and prognostic values are limited.
Damage to endothelial cells results in detachment into the circulation in their entirety or as microparticles. Endothelial microparticles have been found to be increased in vasculitis and atherosclerotic diseases. There is evidence to suggest that endothelial microparticles may contribute directly to endothelial dysfunction. The quantity of circulating endothelial cells and microparticles is therefore a potentially useful marker of endothelial function. With regard to the repair of damaged endothelium, apart from the proliferation of local mature endothelial cells, the importance of bone marrow–derived endothelial stems cells and endothelial progenitor cells is increasingly recognized. Mobilization of endothelial progenitor cells is in part nitric oxide–dependent. Furthermore, the number of progenitor cells has been shown to correlate negatively with the cardiovascular risk score and positively with brachial artery flow-mediated dilation in adults. The usefulness of endothelial microparticles and endothelial progenitor cells as markers of endothelial function in the pediatric population awaits further clarification.
MicroRNAs (miRNAs) are small noncoding single-stranded RNAs that form complementary pairs with specific target messenger RNAs to negatively regulate their expression through translational repression or degradation. The understanding of miRNAs in cardiac and vascular biology has significantly expanded in the past decade. The role of circulating miRNAs as diagnostic and prognostic markers in cardiovascular disease is currently being actively explored. Recently, in obese children, three circulating miRNAs—including hsa-miR-125a-5p, hsa-miR-342-3p, and hsa-miR-365b-3p—have been shown to be potential biomarkers of endothelial dysfunction. The mechanistic link between miRNA expression and endothelial dysfunction remains to be explored, however.
Arterial Structure
Functional alteration of the arterial system with endothelial dysfunction and arterial stiffening is regarded as an early event in the development of cardiovascular disease. Early detection of subclinical alteration of the arterial structure in the young may provide a window for assessment of cardiovascular risk. High-resolution ultrasonography has enabled the interrogation of structural alterations of superficial arteries. The intima-media thickness of the carotid artery is commonly measured and regarded as a surrogate marker of subclinical atherosclerosis. In adults, the associations between carotid intima-media thickening and traditional cardiovascular risk factors and coronary artery disease are well documented. Its predictive value of future cardiovascular events has also been shown. In clinical trials, carotid intima-media thickness is used as an endpoint to assess the impact on cardiovascular risk reduction of pharmacologic interventions.
There is overwhelming evidence to suggest that atherosclerosis begins in childhood. The extent of atherosclerotic changes in children and young adults has been found to correlate with cardiovascular risk factors similar to those identified in adults (see Chapter 25 ). Hence development and application of robust noninvasive methods to assess early atherosclerotic changes of the arteries in the young may improve risk stratification and enable early institution of interventions.
Measurement of Intima-Media Thickness
Recommendations for the standardization of measurement of intima-media thickness in children and adolescents have been summarized in statements made by the American Heart Association and the Association for European Paediatric Cardiology. For imaging of the carotid artery with the aim of measuring intima-media thickness, high-frequency linear array probes with frequencies of 7 MHz or above should be used. Recently the use of ultrahigh-resolution ultrasound, with frequencies in the range of 25 to 55 MHz, has been shown to be feasible and probably more accurate for the measurement of carotid intimal-medial thickness in young children. To ensure optimal imaging of the arterial walls, the carotid artery should be imaged as perpendicular as possible to the plane of ultrasound. Whereas combined assessment of both the near and far walls is common in adult studies, most of pediatric studies have focused on the assessment of the more clearly visualized far wall of the distal 10 mm of the common carotid artery ( Fig. 74.11 ). In several neonatal and pediatric studies, the intimal-medial thickness of the aorta was measured. Manual measurement using online calipers has increasingly been replaced by automatic analysis of acquired B-mode images ( Fig. 74.12 ) or real-time radiofrequency tracking technology.


Systemic Arterial Dysfunction in Childhood
Age-Related Evolution
Aortic, upper limb, and lower limb pulse-wave velocities, as measured by the transcutaneous Doppler technique, increase progressively with age in a cohort of subjects aged 3 to 89 years. Age-dependent increase in brachioradial arterial pulse-wave velocity has similarly been demonstrated in a cohort of children and adolescents aged 6 to 18 years using the photoplethysmographic technique. Analysis of the area under the ascending aortic pressure-time curve using a two-element Windkessel model further reveals a nonlinear increase in total arterial stiffness in children aged 6 months to 20 years.
Previous findings did not, however, suggest that the change in pulse-wave velocity with age is due entirely to differences in systemic blood pressure. Rather, the gradual increase in arterial stiffness with age is probably related to progressive medial degeneration. With cyclic mechanical stress, fragmentation of the elastin fibers and transfer of stress to the stiffer collagen fibers result in a progressive increase in vascular stiffness. Studies of developmental changes in arterial structure during childhood have further demonstrated a progressive increase in intimal and medial thickness after birth. Hence the observed age-related increase in stiffness is likely related to progressive structural changes in the arterial wall during childhood.
In otherwise healthy adults, endothelial function has been shown to deteriorate with aging. Progressive endothelial dysfunction appears to occur earlier in men than in women. Although puberty has been speculated to be a critical period for the vascular endothelium, a recent study showed that endothelial function as assessed by brachial artery flow-mediated dilation does not change significantly during the pubertal period.
Cardiovascular Risk Factors
Childhood obesity is a global epidemic. Obese children have increased stiffness of the abdominal aorta and carotid artery, and endothelial dysfunction is evidenced by elevated serum biomarkers of endothelial activation and impaired brachial artery flow-mediated dilation, which improves with exercise training. Dyslipidemia, diabetes mellitus, low-grade inflammation, and concomitant metabolic syndrome in obese children are linked with arterial dysfunction. Obesity-related peptides exert significant additional influence on the vasculature. Elevations in leptin have been shown to be associated with impaired arterial distensibility in healthy children and in children with type I diabetes. The effect of leptin on endothelial function in humans is, however, controversial. Plasma adiponectin, on the other hand, has been shown to correlate with the vasodilator response of the forearm microcirculation to reactive hyperemia.
Children with heterozygous familial hypercholesterolemia have increased stiffness of the common carotid artery and modification of their aortic elastic properties. Impaired brachial artery flow-mediated dilation has also been shown in these children and in those as young as 6 years of age with familial combined hyperlipoproteinemia. Endothelial dysfunction is most pronounced in those with a positive family history of premature cardiovascular disease. Early statin and antioxidant vitamins C and E therapy may potentially restore endothelial dysfunction in these children toward normal. However, the relationship between flow-mediated dilation and low-density lipoprotein cholesterol levels is controversial. Nonetheless, in a population-based study, total and low-density lipoprotein cholesterol levels were found to relate inversely to brachial artery distensibility, suggesting the possibility that cholesterol levels in the general population during childhood may already be of relevance in the pathogenesis of arterial stiffening.
Children with type 1 diabetes have endothelial dysfunction, which is improved by folic acid. Arterial stiffening in these children is suggested by an increase in augmentation index, which is associated with nitric oxide synthase 3 polymorphism. Offspring of parents with type 2 diabetes have a high risk of developing diabetes and atherosclerotic complications. Studies have shown increased aortic and carotid-radial pulse-wave velocity in normoglycemic adults who are offspring of parents with type 2 diabetes.
Intima-media thickening of the common carotid artery is found in children and adolescents with a parental history of premature myocardial infarction. In adults who are offspring of parents with premature cardiovascular disease, apart from intima-media thickening, impaired endothelial-dependent vasodilation of brachial artery has also been demonstrated.
Habitual physical activity in children aged 5 to 10 years has been shown to correlate positively with flow-mediated dilation, suggesting that its cardiovascular protective effect may be mediated via the endothelium. In another cohort of 10-year-old children, physical activity was found to correlate inversely with arterial stiffness.
Associations between increased baseline high-sensitivity C-reactive protein concentrations and the risks of developing cardiovascular disease in adults have been reported. In healthy children, serum high-sensitivity C-reactive protein concentrations have been found to have an inverse dose-dependent relationship with the magnitude of brachial artery flow-mediated dilation. Increased serum high-sensitivity C-reactive protein levels are found in obese adolescents. Analysis of the 1999 to 2000 National Health and Nutrition Examination Survey showed a strong independent association between body mass index and C-reactive protein level even in young children aged 3 to 17 years. However, in children and adolescents who do not have much of an atherosclerotic burden, whether high-sensitivity C-reactive protein is a risk factor or a risk marker requires further clarification.
In children with homozygous homocystinuria, impaired brachial artery flow-mediated dilation has been demonstrated in those as young as 4 years. In the general pediatric population, findings of elevated homocysteine levels in children with a family history of premature cardiovascular disease are conflicting. Although hyperhomocysteinemia is a risk factor for endothelial dysfunction in middle-aged and elderly subjects, there are no data to suggest a link between arterial dysfunction and serum homocysteine levels in children.
Prenatal Growth Restriction
It has been almost 3 decades since the report of associations between low birth weight and increased risk of cardiovascular disease. These findings, having been replicated in subsequent studies, have formed the basis of the fetal origins hypothesis, later termed developmental origins of disease, which implicates the origin of cardiovascular disease from adaptations to an adverse environment in utero.
There is now substantial evidence that individuals who are born small are at risk of vascular dysfunction in childhood and thereafter. Arterial endothelial dysfunction has been found in term infants, children, and young adults with low birth weight. Another study showed elevated uric acid in children with low birth weight and a graded inverse relationship between uric acid and flow-mediated dilation. In children born at term, leanness at birth has been reported to correlate with the lowest endothelium-dependent microvascular responses and the highest carotid stiffness indexes. In infants with umbilical placental insufficiency before birth, the increase in afterload has been shown to result in a decrease in aortic distensibility during the neonatal period, suggesting an alteration of aortic wall structure. Furthermore, reduced compliance of the aorta and conduit arteries of the legs has been shown to occur in adults born small.
The risk of arterial dysfunction for individuals who are born small as a result of prematurity is controversial. Nonetheless, preterm birth may attenuate the association between low birth weight and endothelial dysfunction. With regard to arterial stiffness, reduced aortic wall distensibility and whole-body compliance have been shown in premature infants with very low birth weight as early as the neonatal period. Other studies have demonstrated inverse relationships between systemic arterial stiffness and gestational age and birth weight standardized for gestational age.
In monozygotic twins with twin-twin transfusion syndrome, peripheral conduit arterial stiffness has been shown to be increased during infancy in the growth-restricted donor twins. Such vascular programming can be ameliorated, albeit not completely abolished, by intrauterine endoscopic laser ablation of placental anastomoses. Even in monozygotic twins without twin-twin transfusion syndrome, the twin with the lower birth weight has been found to have a higher systolic blood pressure and pulse pressure and impaired endothelial function in childhood.
The mechanism whereby low birth weight is associated with increased arterial stiffness in childhood and adulthood remains unclear. The reported endothelial dysfunction in individuals born preterm and small-for-gestational age suggests that functional alteration of arterial tone may contribute to an increase in systemic arterial stiffness. Preferential perfusion of the upper part of the body in intrauterine growth retardation may affect the mechanical properties of the large arteries. Hence selective carotid arterial atherosclerosis has been found to be more severe in elderly people with the lowest birth weight. Another proposed mechanism is the impairment of synthesis of elastin in the arterial wall. Superimposed circulatory imbalance, as happens in the donor twins in twin-twin transfusion syndrome, probably acts synergistically with growth restriction to cause the vascular programming, although the exact mechanism remains to be defined. The exact mechanism of endothelial dysfunction in individuals with low birth weight is even more elusive.
Nutritional Issues
The association between early growth restriction and arterial dysfunction highlights the potential importance of nutrition in antenatal and early postnatal life on long-term vascular programming. In a recent mother-offspring study, higher consumption of oily fish by mothers in late pregnancy has been associated with lower aortic pulse-wave velocity measured in their children at the age of 9 years. Leeson et al. studied the relation between duration of breastfeeding and brachial artery distensibility in a young adult population-based cohort. They found an inverse relation between duration of breastfeeding and arterial distensibility even after adjusting for current lipid profile, body mass index, and social class. A study in 10-year-old children also demonstrated a positive association between the duration of breastfeeding and stiffness of the aortofemoral arterial segment as determined by pulse-wave velocity. On the other hand, data from the Cardiovascular Risk in Young Finns Study suggest that adult men who had been breast-fed have better brachial endothelial function compared with those who had been formula-fed. It is important to realize, however, that there is to date little consistent evidence that breastfeeding influences subsequent mortality related to cardiovascular disease and that the current advice on breastfeeding practice has not been altered by these findings.
In children receiving long-term parenteral nutrition, a significant increase in the elastic modulus of the common carotid artery and impairment of brachial artery flow-mediated dilation have been demonstrated. Although the mechanisms are unclear, animal studies suggest that the infusion of lipid emulsions or high-concentration dextrose can cause endothelial damage and vascular remodeling. It remains unknown whether vascular dysfunction is reversible after the reestablishment of enteral feeding.
Childhood Vasculitides
Kawasaki disease, a childhood vasculitis of unknown etiology, is the most common cause of acquired heart disease in children in developed countries (see Chapter 53 ). The inflammatory damage to coronary and other medium-sized arteries in the acute phase of the disease is well described. Long-term structural alteration and functional disturbance of the coronary arteries are also clearly documented. Increasingly, systemic arterial dysfunction is recognized in children with a history of Kawasaki long after the acute illness.
Impaired brachial artery flow-mediated dilation has been demonstrated in patients, even in those without early coronary arterial involvement, studied at a median of 11 years after the acute illness. Intravenous administration of vitamin C may improve the impaired flow-mediated dilation. An increase in circulating endothelial cells has also been found in patients with and without coronary artery aneurysms years after the acute illness. Increased stiffness of the carotid and brachioradial arteries occurs in a dose-dependent manner to the degree of coronary arterial involvement. This corroborates the findings of reduced characteristic impedance and total peripheral arterial compliance in Kawasaki patients regardless of persistence of coronary artery aneurysms. Additionally, the mannose-binding lectin genotype and polymorphisms of the C-reactive protein and tumor necrosis factor-α genes may exert a modulating effect on arterial stiffness in the long term in these patients.
Chronic low-grade inflammation, as reflected by elevated high-sensitivity C-reactive protein, in patients with coronary aneurysm formation has been associated positively with carotid arterial stiffness. There is histologic evidence of fibrointimal thickening and infiltration of lymphocytes and plasma cells in the coronary arterial walls in fatal cases of Kawasaki disease years after the apparent resolution of vascular inflammation and in the absence of early detectable coronary arterial abnormalities. Chronic activation of the monocyte chemoattractant protein-1/chemokine receptor CCR2 pathway and inducible nitric oxide synthase may play a role in this chronic low-grade inflammatory process. Positron emission tomography scanning provides in vivo evidence of ongoing inflammation of giant coronary and axillary aneurysms. This vascular inflammatory remodeling may be dampened by the use of statins.
Marked acceleration of atherosclerosis is evident in a mouse model of Kawasaki disease. Although cross-sectional studies of carotid intima-media thickening in patients with Kawasaki disease have been conflicting, the finding of an increase in carotid intima-media thickening in patients with persistent coronary aneurysms appears to be a more consistent one. In the only longitudinal study performed to date, Dietz et al. found an initial increase but with subsequent normalization of carotid intima-media thickness in patients without coronary aneurysms. On the other hand, patients with giant coronary aneurysms showed a trend toward persistent increase in carotid intima-media thickness. Whether this long-term structural change of the artery represents premature atherosclerosis or a distinct type of vasculopathy continues to be a matter of debate.
Limited data exist for systemic arterial dysfunction in other types of childhood vasculitis. Transient impaired of forearm vascular endothelium-dependent relaxation has been found in children during the acute phase of Henoch-Schönlein purpura. In children with polyarteritis nodosa, a chronic vasculitis characterized by recurrent episodes of inflammatory exacerbation, stiffening of the brachioradial artery with amplification during episodes of inflammatory exacerbation has been demonstrated. Endothelial microparticles have also been found to be significantly increased in children with systemic vasculitis and to correlate with disease activity score.
Vasculopathies in Syndromal Disorders
Patients with Marfan syndrome have increased aortic stiffness, as evidenced by the decreased distensibility and increased stiffness indexes, increased pulse-wave velocity, and decreased tissue Doppler-derived systolic and diastolic velocities of the aortic wall. The correlation between fibrillin-1 genotype and aortic stiffness in these patients is, however, poor. Endothelial dysfunction in Marfan syndrome may be related to a defective role of subendothelial fibrillin in endothelial cell mechanotransduction and downregulation of signaling of nitric oxide production, which contributes further to arterial stiffening. Importantly, aortic stiffness has been shown to be an independent predictor of progressive aortic dilation and aortic dissection. β-Blocker therapy, angiotensin-converting enzyme inhibition, and losartan appear to reduce aortic stiffness, which may in turn slow aortic dilation and delay aortic root replacement, although robust data remain elusive. In children and young adults with Marfan syndrome, Loeys-Dietz syndrome, and Ehlers-Danlos syndrome, the increase in aortic stiffness is associated with a higher rate of aortic root dilation and surgical root replacement on follow-up.
Haploinsufficiency of the elastin gene is implicated in the arteriopathy of Williams syndrome. Despite a biologic basis for abnormal elastic fibers, the results of studies exploring arterial elastic properties in patients with Williams syndrome are controversial. Studies have shown increased stiffness of the ascending aorta and aortic arch and implied that abnormal mechanical property of the arteries may contribute to systemic hypertension commonly found in patients with Williams syndrome. By contrast, paradoxical reduction of stiffness of the common carotid artery has also been reported.
Systemic arterial abnormalities—including bicuspid aortic valve, coarctation of the aorta, and aneurysmal dilation of the aortic root—are common in Turner syndrome. Although histologic evidence of cystic medial necrosis has been reported in Turner syndrome, these findings are not consistently present. Functionally, the carotid augmentation index has been found to be increased, explainable in part by the short stature, whereas carotid-femoral pulse-wave velocity and brachial artery flow-mediated dilation remain normal in these patients. It is worthwhile to note, however, that an isolated bicuspid aortic valve is associated with progressive dilation of the ascending aorta in both children and adults and with increased aortic stiffness.
Congenital Heart Disease
Aortic medial abnormalities with elastic fiber fragmentation have been found in intraoperative biopsies and necropsy specimens of a variety of congenital heart disease in patients ranging from neonates to adults. These congenital heart lesions include tetralogy of Fallot with or without pulmonary atresia, common arterial trunk, complete transposition of the great arteries (TGA), coarctation of the aorta, double-outlet ventricles, and univentricular hearts. Whether these abnormalities are inherent or acquired is, however, unknown.
In tetralogy of Fallot, these intrinsic histologic abnormalities have been implicated in the pathogenesis of progressive aortic root dilation despite surgical repair. In children and adults with repaired tetralogy of Fallot, increased aortic stiffness and reduced aortic strain have been documented and found to be related to aortic root dimensions. Preferential stiffening of the central over peripheral conduit arteries and a marked increase in the ascending but not descending aortic stiffness have been demonstrated. Furthermore, the heart-femoral pulse-wave velocity and carotid augmentation index were found to be significant determinants of the size of sinotubular junction, suggesting that central arterial stiffening may contribute to progressive aortic root dilation in these patients. The fibrillin-1 gene variant has also been associated with an increased risk of aortic dilation in patients with repaired tetralogy of Fallot.
In complete TGA, abnormal aorticopulmonary septation has been hypothesized to be associated with events in elastogenesis. In patients undergoing two-stage anatomic correction, decreased distensibility of the neoaorta has been thought to be related to pulmonary arterial banding. Nonetheless, even after a one-stage arterial switch operation, impaired distensibility of the neoaorta has similarly been found. Furthermore, reduced distensibility of the thoracic aorta at the level of the isthmus in addition to the neoaortic root has been documented. Upon submaximal bicycle stress testing, persistent elevation of aortic stiffening beyond the normal physiologic changes has been associated with a higher systolic blood pressure during exercise. Previous studies have also documented an increase in stiffness index of the carotid artery in patients after either atrial or arterial switch operations, suggesting that impaired elastogenesis may be an intrinsic component of this congenital anomaly.
Structural abnormalities of the aortic segment proximal to the site of aortic coarctation are characterized by an increase in collagen and a decrease in smooth muscle content. The finding of an increased ascending aortic stiffness index in neonates with coarctation before surgery supports that impairment of elastic properties of the prestenotic aorta is a primary abnormality. Despite successful surgical repair, functional impairments—including impaired flow-mediated dilation, reduced nitroglycerine-induced vasodilation, and increased pulse-wave velocity of conduit arteries proximal to the site of coarctation—persist. Distensibility of the aortic arch has also been shown to be significantly lower than that of the distal thoracic aorta. Enhanced aortic pressure-wave reflection has been attributed to a major reflection point at the site of coarctation repair. The finding of an inverse relationship between the magnitude of the brachial artery vasodilation response to nitroglycerine and 24-hour systolic blood pressure implicates a possible role of reduced vascular reactivity in the development of systemic hypertension and left ventricular hypertrophy in patients despite successful repair of coarctation. The importance of early coarctation repair for the possible prevention of late vascular dysfunction is highlighted by the inverse relationships between age at repair and stiffness and vascular reactivity of the precoarctation arterial segments.
In Fontan patients, magnetic resonance angiographic assessment has revealed a prevalence of 8.5% and 22% of severe dilation of the aortic root and ascending aorta, respectively. Importantly, dilation of the ascending aorta has been associated with an increase in the aortic stiffness index. Increased central and peripheral arterial augmentation indexes have also been found in Fontan patients. Functionally, in adolescents and young adults, worse indexes of endothelial function and arterial stiffness are associated with lower exercise capacity, cardiac output, and physical activity and worse quality-of-life parameters.
Endothelial dysfunction has also been associated with congenital cardiac anomalies. In adults with cyanotic congenital heart disease, impaired forearm blood flow response to the intra-arterial infusion of acetylcholine has been shown. The cause is probably multifactorial, being related to reduced nitric oxide production, hypoxemia, and secondary erythrocytosis. Altered levels of biomarkers of endothelial activation have been shown in a small cohort of children and adolescents with univentricular hearts who have undergone cavopulmonary connection. In patients after the Fontan operation, reduced brachial artery flow-mediated dilation and, in a subset, impaired nitroglycerine-induced vasodilation have been demonstrated. A negative correlation, albeit weak, was found in post-Fontan patients between flow-mediated dilation and serum levels of the nitric oxide pathway inhibitors asymmetric and symmetric dimethlyarginine. Furthermore, patients on angiotensin-converting enzyme inhibitors tended to have better endothelial function. Nonetheless the usefulness of angiotensin-converting enzyme inhibitors in the post-Fontan state remains debatable.
Systemic Diseases
Rheumatoid arthritis and systemic lupus erythematosus provide clinical models for determining the relationship of chronic systemic inflammation to arterial dysfunction. In children and young adults with juvenile idiopathic arthritis, reduced arterial elasticity, increased aortic pulse-wave velocity, decreased flow-mediated dilation, and increased carotid intima-media thickness have been reported. In an interventional study, the use an antiinflammatory medication for a year has been shown to decrease carotid intima-media thickness. Few studies have evaluated arterial function in children with systemic lupus erythematosus. In women with systemic lupus erythematosus, brachial artery flow-mediated dilation has been found to be impaired and carotid arterial stiffness increased. In adolescents and young adults with lupus of pediatric onset, endothelial function has been found to be normal. However, another study demonstrated increased carotid artery stiffness associated with left ventricular hypertrophy and subclinical left ventricular dysfunction.
Acute exposure to extrinsic inflammatory insult in children who had an acute infection or are convalescing from an infection in the previous 2 weeks has also been shown to cause transient impairment of brachial artery flow-mediated dilation. The endothelial dysfunction was found to recover in most but not all of the children studied at follow-up 1 year later. Possible mechanisms include a direct effect of virus or an indirect effect through inflammatory cytokines on endothelial function. Apart from common acute childhood infections, chronic infection with human immunodeficiency virus in children has been associated with arterial dysfunction. The functional alteration is characterized by impaired brachial artery flow-mediated dilation and increased elastic modulus of the carotid arterial wall. Additionally, endothelial dysfunction was found to be more pronounced in children receiving protease inhibitor therapy.
In adults with chronic renal failure, premature atherosclerosis is a major cause of morbidity and mortality. There is evidence to suggest that children with end-stage renal failure are similarly predisposed to an increased risk of cardiovascular disease. Increased aortic pulse-wave velocity and carotid artery stiffness are independent predictors of cardiovascular mortality in adults with end-stage renal failure. In children on hemodialysis, increased carotid-femoral pulse-wave velocity and augmentation index have also been demonstrated. Even after successful pediatric renal transplantation, carotid artery stiffness remains elevated and is associated with a higher daytime systolic blood pressure load and receipt of cadaveric kidney. Apart from arterial stiffening, endothelial dysfunction has been demonstrated in children with chronic renal failure even in the absence of classic cardiovascular risk factors and in those after transplantation. Limited evidence suggests that oral folic acid, but not L-arginine, may improve endothelial function in children with chronic renal failure by lowering the homocysteine level and increasing the resistance of low-density lipoprotein to oxidation.
Increased iron storage has been linked with the risk of atherosclerosis. Iron overloading in patients with β-thalassemia major results in alterations of arterial structures with disruption of elastic tissue and calcification. In adolescent and adult patients with β-thalassemia major, increased stiffness of the carotid artery, brachioradial artery, and aorta has been shown in vivo. Importantly, systemic arterial stiffening is inversely related to brachial artery flow-mediated dilation and positively with left ventricular mass and carotid intima-media thickness. Furthermore, elevated pulsatile and static afterload was found in patients with β-thalassemia major. Apart from oxidative damage related to iron overload, the cell-free hemoglobin in hemolytic disease has also been implicated in mediating vascular dysfunction by limiting the availability of nitric oxide.
Sleep-related disorders are common in children and may affect arterial function. In children with primary snoring, higher daytime systemic blood pressure, increased brachioradial artery stiffness, and reduced flow-mediated dilation of the brachial artery independent of obesity have been reported. In adults with obstructive sleep apnea, increased carotid-femoral pulse-wave velocity and brachial-ankle pulse-wave velocity have been reported. Pediatric data in this regard are lacking. On the other hand, nonobese children with obstructive sleep apnea were found to have endothelial function, which could be reversed after adenotonsillectomy. Children with obstructive sleep apnea and altered endothelial function have been found to have reduced circulatory endothelial progenitor cells. On the other hand, the levels of circulating microparticles were found to be increased and, in particular, platelet-derived microparticles were found to be associated with vascular dysfunction. Recent studies also suggest possible involvement of a triggering receptor expressed on myeloid cells-1, pentraxin-3, adropin, and nicotinamide adenine dinucleotide phosphate oxidase in causing endothelial dysfunction in children with obstructive sleep apnea.
In children with mitochondrial myopathy, encephalopathy, lactic acidosis, and stroke (MELAS), the level of l -arginine has been found to be significantly lower during stroke-like episodes and associated with reduced brachial artery flow-mediated dilation. Prolonged l -arginine supplementation has been shown to reduce the stroke-like episodes and to normalize endothelial dysfunction.
In vitro studies have demonstrated the toxic effects of anthracyclines on endothelial cells. Finally, in survivors of childhood cancers, increased aortic stiffness and reduced carotid distensibility have recently been reported.
Genetic Considerations
The genetic aspect of arterial stiffness is increasingly being unveiled. The phenomenon of arterial stiffening in genetic syndromes associated with the mutation or deletion of genes encoding structural proteins of the arterial wall has been alluded to earlier. Studies have demonstrated moderate to substantial heritability of common carotid artery stiffness, augmentation index, and carotid-femoral pulse-wave velocity in different ethnic populations. Genome-wide association studies, linkage analysis, and candidate gene polymorphism association studies have revealed different groups of genes that may contribute to arterial stiffening. These genes are involved in the renin-angiotensin-aldosterone system, matrix components and metalloproteinases, and the nitric oxide pathway. Other important gene variants include β-adrenergic receptors, endothelin receptors, and inflammatory molecules, which are implicated in the pathophysiology of arterial stiffness. Genome-wide association studies have identified additional genes that have no apparent relationship to mechanisms of arterial stiffness but which may target transcriptional pathways controlling gene expression, differentiation of vascular smooth muscle cells, apoptosis of endothelial cells, or the immune response within the vascular wall. Detailed discussion on the genetics of arterial stiffness is beyond the scope of this chapter; however, interested readers are referred to recently published reviews.
The genetics of endothelial dysfunction are less clear. Potential genetic contribution is evidenced by studies demonstrating that young individuals with a family history of cardiovascular disease have impaired endothelial function and that polymorphisms of angiotensin-converting enzyme and endothelial nitric oxide synthase genes may influence endothelial function. In children, endothelial dysfunction is found in single-gene disorders including homocystinuria and familial hypercholesterolemia. In a large community-based sample, the estimated heritability of brachial artery flow-mediated dilation has been shown to be modest. Although polymorphisms of genes encoding factors involved in the regulation of nitric oxide synthesis have been implicated in endothelial dysfunction, further studies are warranted before any conclusions can be drawn.
Clinical Implications
The prognostic implications of arterial dysfunction in adults have been alluded to earlier. Whether arterial stiffening and endothelial dysfunction represent genuine cardiovascular risk factors and can predict future cardiovascular events in children and adolescents is unclear. Nevertheless it appears unrealistic to assess the prognostic value of indexes of arterial function in childhood only in terms of endpoints as cardiovascular morbidity and mortality. The use of carotid intima-media thickness (see earlier) as a structural surrogate measure of atherosclerosis is a potentially useful alternative. Increased carotid intima-media thickness is documented in several of the aforementioned pediatric conditions including childhood obesity, familial hypercholesterolemia, type 1 diabetes, hypertension, metabolic syndrome, family history of premature myocardial infarction, children born small for gestational age, children infected with human immunodeficiency virus on antiretroviral therapy, Kawasaki disease, chronic renal disease, and thalassemia major.
Early identification of arterial dysfunction that potentially precedes and induces atherosclerotic changes provides a window for early intervention. The potential beneficial effects on endothelial function of folic acid in children with renal failure, antioxidant vitamins and statins in those with familial hypercholesterolemia, vitamin C and statins in those with Kawasaki disease, and exercise training in obese children have been discussed. Furthermore, in patients with Marfan syndrome, β-blocker therapy, and angiotensin-converting enzyme inhibition, and losartan appear to reduce aortic stiffness, albeit with uncertain effects on aortic dilation itself. However, intuitive longitudinal studies are required to determine whether therapeutic intervention to improve arterial function will be translated into clinical benefits or whether lifestyle and dietary modifications in healthy children may prevent arterial dysfunction in later life. Nonetheless this is a rich area for research, with potentially huge benefits at an individual and societal level.
Given that the systemic arterial system receives and distributes output from the systemic ventricle, satisfactory performance of the systemic ventricular pump depends not only on its intrinsic properties but also on its optimal interaction with the systemic circulation. Dysfunction of either of the components of the cardiovascular system would inevitably affect performance of the other. This issue of ventriculoarterial interaction is discussed in the following section.
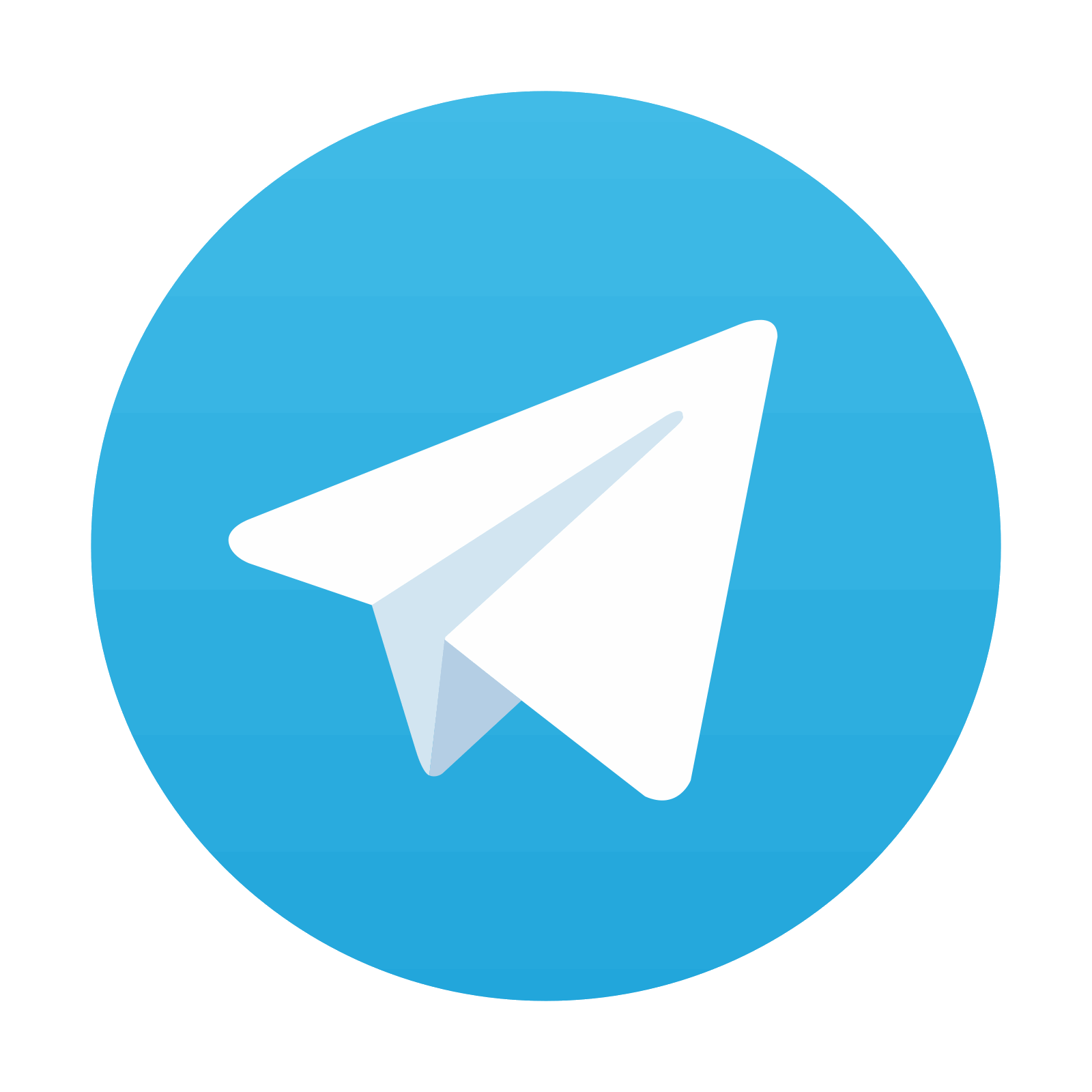
Stay updated, free articles. Join our Telegram channel
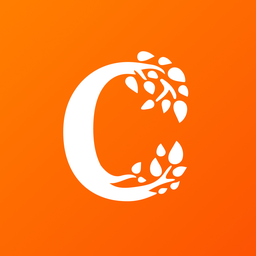
Full access? Get Clinical Tree
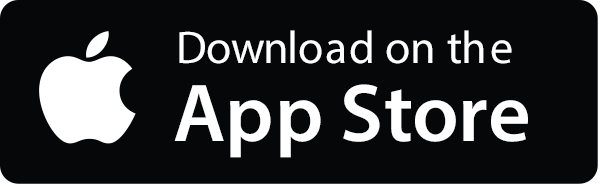
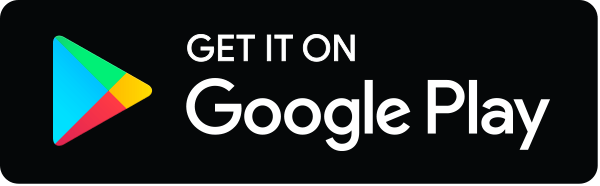