The systemic circulation refers to the circulation in which blood is carried from the systemic ventricle, which is the left ventricle in the setting of ventriculo-arterial concordance, through a network of arteries and arterioles to the tissue capillaries, and drained via the systemic venous system to the systemic venous atrium.
The systemic arterial system serves two important functions. First, it acts as a low-resistance conduit through which blood is distributed to different parts of the body. Second, the arterial tree buffers the pulsatile pressure to convert systemic ventricular pulsatile ejection into a steady stream of capillary flow. Additionally, the endothelium, which lines the vascular lumen, exerts important vascular homeostatic effects through production of a variety of substances. Hence, alterations of the mechanical properties of the arterial wall and function of the endothelium have significant implications on normal functioning of the systemic arterial system. Furthermore, optimal performance of the systemic ventricle depends on its favorable interaction with the systemic circulation. In the setting of congenital heart disease, the systemic ventricle may be a morphological left ventricle, morphological right ventricle, or single functional ventricular chamber of right, left or indeterminate morphology. As a result of systemic arterial dysfunction, an unfavorable ventriculo-arterial interaction may result. On the other hand, systemic ventricular dysfunction may also predispose to systemic arterial dysfunction.
In this chapter, the systemic circulation is discussed from structural, physiological, and mechanical perspectives. Measures of arterial function and paediatric conditions associated with systemic arterial dysfunction are then highlighted. Finally, the concept of ventriculo-arterial interaction and its relevance in congenital and acquired heart disease in the young are described. The systemic venous system is discussed in Chapter 23 .
SYSTEMIC ARTERIAL SYSTEM
Structure
The systemic arterial tree begins with the aorta, the largest arterial trunk that arises from the left ventricle. The aorta ramifies into tributaries to perfuse all parts of the body, with the exception of hair, nails, epidermis, cartilage, and cornea. The large central arteries are protected within the thoracic and abdominal cavities, while peripheral conduit arteries run along the flexor surfaces in the upper and lower limbs where they are less exposed to injury. The fashion in which the arterial tree ramifies varies: an arterial trunk may give off several branches in succession and continue as a main trunk, give off a short trunk that subdivides into several branches, or bifurcate at its distal end. The ascending aorta arises at the base of the left ventricle and gives off the first branches, the right and left coronary arteries. It continues as the aortic arch, from which the brachiocephalic, left common carotid, and left subclavian arteries arise. The thoracic descending aorta begins as a continuation of the aortic arch and penetrates the diaphragm to continue as the abdominal descending aorta. The celiac trunk and superior and inferior mesenteric arteries arise from the abdominal descending aorta to supply the liver and gastrointestinal tract, while the renal arteries branch off at right angles to perfuse the kidneys. The descending aorta bifurcates at its distal end into the right and left common iliac arteries, the latter bifurcating into the internal iliac artery to supply the pelvic organs and the external iliac artery that continues as the femoral artery to supply the lower limbs. The systemic arterial tree is a tapering branching system. Hence, the aorta tapers from its origin to its termination at the iliac bifurcation and branched daughter vessels are always narrower than the parent vessel, which has implications on wave reflection. The arterial ramifications end in arterioles, which then usually continue as capillaries. Beyond the major arterial branches, the total cross sectional area increases progressively to the capillary bed. Apart from size, the proportion of cellular and structural components also varies along the arterial tree. Notwithstanding these variations, the arterial wall is made up of three constant layers: an internal tunica intima, a tunica media, and an external tunica adventitia.
The intima comprises the endothelium, a subendothelial layer, and an elastic membrane. The endothelium consists of a monolayer of cells that line the vascular lumen. Apart from forming a physical barrier between the circulating blood components and the vascular wall, the endothelial cells play a pivotal role in vascular homeostasis. The subendothelial layer is made up of fibroblasts and variable amount of collagen. The internal elastic membrane consists of a network of elastic fibres and forms the boundary with the media.
The media, usually the thickest layer in the arterial wall, is responsible for the mechanical properties of the vessel. Its structural components are vascular smooth muscle cells and extracellular matrix, the latter consisting of elastic lamellas, collagen fibres, structural glycoproteins, and ground substance. 1 While vascular smooth muscle cells maintain vascular tone through contraction and relaxation, the extracellular matrix of the media provides a structural framework for optimal functioning of the blood vessels.
The elastic fibres in the media, arranged in concentric lamellas that form boundaries between layers of vascular smooth muscle cells, are 90% represented by elastin. Cross-linking of elastin confers to the arteries elasticity, the ability to distend during cardiac systole and recoil during diastole. Elastin has also further been implicated in the control of proliferation and phenotype of smooth muscle cells. 2 Elastin has an estimated half-life of more than 40 years in humans, and the rate of its synthesis is thought to be negligible in adulthood. 3–5 Hence, it appears that damaged elastin, as a result of either degeneration or pathological process, is unlikely to be replaced. Other constituents of elastic fibres include microfibrillar-associated glycoproteins and fibrillin. 6–8 Fibrillin forms a microfibrillar network that serves as scaffolding for deposition of elastin and assembly of elastic fibres. A recently discovered protein, fibulin-5, also plays a critical function during elastic fibre development through its interactions with elastin and integrins. 9,10 Other structural glycoproteins in the arterial wall include fibronectin, vitronectin, lamin, entactin/nidogen, tenascin, and thrombospondin. 11,12
Collagens are composed of three polypeptide α-chains arranged to form a triple helix, which confers tensile strength to the vessel wall. Types I and III collagen are the major fibrillar collagens in blood vessels, constituting about 90% of vascular collagens. 13 Collagen is the stiffest component of the arterial wall, with an elastic modulus of 10 8 to 10 9 dyne/cm 2 14 By contrast, the elastic modulus of elastin is of the order of 10 6 dyne/cm. 2,15,16 Hence, the absolute and relative quantities of elastin and collagen contribute significantly to stiffness of the arterial wall. The elasticity of the arterial wall is a non-linear function of transmural pressure. To explain the non-linear elasticity, a qualitative model proposes that at low pressure, the tension is borne by elastin, and as the pressure and stretch increase, collagen fibres take on an increasing fraction of the tension with progressive stiffening of the blood vessel, hence preventing its over-distention at high pressure. 17 Indeed, increasing recruitment of collagen fibres in the human brachial artery as transmural arterial pressure increases has been shown in vivo. 18 Recent proposed models take into account the contribution of vascular smooth muscle cells, viscoelastic properties of the matrix proteins, residual stresses due to growth and remodeling, and gradual recruitment of collagen fibres with increasing pressure. 19–22
The ground substance is filled by proteoglycans. Proteoglycans are macromolecules that possess one or more linear glycosaminoglycan chains linked to a core protein. The proteoglycans in the vessel wall are hyaluronan, versican, biglycan, decorin, lumican, syndecans, fibroglycan, and glypican. 23 The proteoglycans have diverse roles in the organisation of connective tissue structure, regulating cellular activities and metabolism, permeability, filtration, and hydration, and controlling cytokine biodisponibility and stability. 24–27 Different components of the extracellular matrix can be degraded by matrix metalloproteinases. Importantly, matrix metalloproteinases play a fundamental role in the degradation of vascular extracellular matrix 28 not only during normal physiological vascular remodeling, but also during pathological remodeling. 23,29
The distribution of structural components within the media varies along the arterial tree, hence accounting for the difference in mechanical properties between proximal and distal arteries. 30 Of significance is the fall in elastin to collagen ratio and increase in smooth muscle cells with increasing distance from the heart. 31,32 Arteries have been categorised as elastic or muscular based on structural composition of the media. Hence, the aorta and its major branches are described as elastic arteries, while brachial and femoral arteries are regarded as muscular conduit arteries. At the arteriolar level, the media consists of essentially one to several layers of smooth muscle cells. Thus, the basic architecture of arteries justifies the division of the systemic arterial tree into a proximal compartment, in which elastin predominates, and a distal compartment, in which collagen and vascular smooth muscle cells predominate. 30 Alterations of structural components of the media as a result of degeneration, genetic mutations, or imbalanced activities of metalloproteinases and their inhibitors can have significant impact on the mechanical properties of the vessels.
The adventitia contains mainly fibroblasts and collagen fibres and some elastic fibres. Increasingly, the contribution of adventitial layer to the elastic properties of arteries is recognised. 33,34 Nutrient vessels, vasa vasorum, arise from a branch of the artery or from a neighbouring vessel to ramify and distribute to the adventitial layer.
Endothelial Function
The endothelium comprises a monolayer of endothelial cells that lines the vascular lumen. It is strategically located between circulating blood components and vascular smooth muscle cells to exert a pivotal role in vascular homeostasis. By producing a wide variety of substances, the endothelium regulates vascular tone, inhibits smooth muscle cell proliferation and migration, controls cellular adhesion, regulates inflammation, and exerts fibrinolytic and antithrombotic actions. In recent years, the concept of endothelial function has extended from the vascular lumen to the vascular wall and adventitia, which are supplied by vasa vasorum considered as an active intravascular microcirculation. 35,36
Nitric oxide, initially identified as the endothelium-derived relaxing factor, 37 is the major vasodilating substance released by the endothelium. Nitric oxide is synthesised from l -arginine by the action of endothelial nitric oxide synthase, primarily in response to sheer stress produced by blood flow. 38 Cofactors including tetrahydrobiopterin and nicotinamide adenine dinucleotide phosphate are involved in nitric oxide production. 39 Apart from shear stress, endothelial nitric oxide synthase can also be activated by bradykinin, adenosine, vascular endothelial growth factor, and serotonin. 40 Asymmetrical dimethylarginine, on the other hand, is an endogenous inhibitor of nitric oxide synthase 41 and has been implicated in the mediation of adverse effects of traditional risk factors on endothelial vasodilator function. 42 Nitric oxide has a half-life of a few seconds in vivo. It diffuses from endothelial cells to exert its relaxation effects on vascular smooth muscle cells by activation of guanylate cyclase, which in turn increases production of cyclic guanosine monophosphate and leads to reduction of intracellular calcium concentration. Apart from regulation of vascular tone through vasodilation, nitric oxide also mediates other important vascular homeostatic functions by exerting inhibitory effects on vascular smooth muscle proliferation, 43 counteracting leucocyte adhesion to the endothelium 44,45 and inhibiting platelet aggregation. 46
The endothelium also mediates hyperpolarisation of the vascular smooth muscle to cause relaxation. 47,48 Although the identity of the endothelium-derived hyperpolarizing factor remains elusive, its hyperpolarizing mechanism is in general considered to be mediated by calcium-activated potassium channels on vascular smooth muscle. 49–52 Candidates of endothelium-derived hyperpolarizing factor include epoxyeicosatrienoic acids, 53,54 potassium ion, 55 gap junctions, 56 and hydrogen peroxide. 57 In human forearm circulation, endothelium-derived hyperpolarizing factor appears to be a cytochrome P-450 derivative, possibly an epoxyeicosatrienoic acid, 58 and contributes to basal vascular resistance and vasodilation evoked by substance P and bradykinin. 59 There is a suggestion that endothelium-derived hyperpolarizing factor might play a compensatory role for the loss of nitric-oxide mediation vasodilation in patients with heart failure. 59,60 Other endothelium-derived vasodilators include prostacyclin and bradykinin. Prostacyclin is produced via the cyclo-oxygenase pathway and acts independently of nitric oxide to cause vasodilation. 61 It also acts synergistically with nitric oxide to inhibit platelet aggregation. Prostacyclin appears to have a limited role in humans in the control of vascular tone. Bradykinin stimulates release of nitric oxide, prostacyclin, and endothelium-derived hyperpolarizing factor.
Regulation of vascular tone by the endothelium is accomplished not only by release of vasodilators, but also by the control of vasoconstrictor tone through release of endothelin 62 and conversion of angiotensin I to angiotensin II at its surface. 63 Endothelin-1, the predominant endothelin isoform in the cardiovascular system, binds to ET A receptors on vascular smooth muscle cells to cause vasoconstriction. 64 At lower concentration, however, endothelin-1 causes transient vasodilation in human forearm circulation, 65 probably due to stimulation of release of nitric oxide and prostacyclin via ET B receptors located on endothelial cells. 66
Vascular Smooth Muscle Function
The primary function of the vascular smooth muscle cells is contraction, during which the cells shorten to reduce vessel diameter, alter vascular tone, and regulate blood flow. This contractile phenotype of vascular smooth muscle cells is characterised by expression of genes that encode contractile proteins, ion channels, and other molecules involved in contraction. 67,68 Excitation-contraction coupling is the process by which cellular signaling pathways modulate activities of ion channels in the smooth muscle sarcolemma, thereby causing alterations in intracellular calcium signaling and other signaling cascades and resulting in contraction or relaxation of vascular smooth muscle cells. The regulation of smooth muscle contraction in vivo is primarily by pharmacomechanical and electromechanical activation of the contractile proteins myosin and actin. 69 Pharmacomechanical coupling refers to activation of contraction by ligands of cell surface receptors without obligatory change in plasma membrane potential. In vascular smooth muscle, the phosphoinositide signaling cascade is the common secondary messenger system utilised by the surface receptors. Electromechanical coupling, on the other hand, involves alterations in plasma membrane potential. Receptor activation may induce an activation of receptor-operated or voltage-dependent channels and lead to passive influx of calcium down its concentration gradient. Detailed discussion of the molecular mechanisms of smooth muscle contraction is beyond the scope of this chapter. Interested readers are referred to several recently published reviews. 70–74 The balance between force generation and release is responsible for the maintenance of vascular tone, 71 which can be envisaged as the sum of forces generated in the vessel wall to oppose the increase in vessel diameter. The vascular tone is influenced by local metabolic substances, humoral factors, and activity of the autonomic nervous system. In the smallest arteries and arterioles, contraction of vascular smooth muscle causes large reduction in the vascular lumen and increases peripheral vascular resistance. In large elastic and muscular conduit arteries, the change in vascular tone is accompanied by an increase in elastic modulus, and hence stiffness, of the arteries.
Apart from a contractile phenotype, vascular smooth muscle cells exhibit other phenotypes. This phenotypic diversity plays an important role in normal development, repair of vascular injury, and vascular disease process. 75,67 Hence, after vascular injury, phenotypic modulation of vascular smooth muscle cells causes upregulation of genes required for their proliferation and production of extracellular matrix and suppression of genes that characterise the contractile phenotype. On the other hand, inappropriate pathological differentiation into other mesenchymal lineages as osteoblastic, chondrocytic, and adipocytic ones may contribute to vessel calcification, altered matrix production, and abnormal lipid accumulation, respectively. 76–80 Recent studies have focused on the understanding of mechanisms that underlie the physiological control and pathologic alterations of phenotypic switching of vascular smooth muscle cells. 67,75,81
Control of Circulation
The regulation of circulation aims to adjust precisely the blood flow in relation to tissue needs and to maintain adequate driving pressure to perfuse the various body tissues. Such control is achieved through local mechanisms, humoral factors, and neural regulation.
Local Control
Auto-regulation refers to the ability to maintain relatively constant blood flow in response to acute changes in perfusion pressure. The coronary, renal, and cerebral circulations exhibit auto-regulation. Two theories have been proposed for this auto-regulatory mechanism. The metabolic theory 82 suggests that elevated perfusion pressure increases blood flow, and hence oxygen delivery and removal of vasodilators, thereby leading to vasoconstriction and reduction of blood flow and vice versa. The myogenic theory 83 proposes that stretching of vascular smooth muscle cells by the elevated perfusion pressure increases their tension, which in turn causes vasoconstriction and reduces the blood flow back to normal. Conversely, less stretching at low pressure leads to smooth muscle relaxation and increased blood flow. The physiological relevance of myogenic constriction lies in its influence on peripheral vascular resistance, provision of vascular tone, and contribution to control of capillary pressure. However, the exact mechanisms that link intraluminal pressure generation to myogenic constriction remain uncertain. 84
Metabolic mechanisms also contribute to the control of local blood flow. Two theories have likewise been proposed. The vasodilator theory proposes that vasodilator substances are formed and released from tissues when metabolic rate increases or oxygen and other nutrient supplies decrease. Suggested vasodilator substances include adenosine, carbon dioxide, potassium ion, hydrogen ion, lactic acid, histamine, and adenosine phosphate. The nutrient theory suggests that blood vessels dilate naturally when oxygen or other nutrients are deficient. Hence, increased metabolism causes local vasodilation by increased utilisation of oxygen and nutrients, a phenomenon known as active hyperaemia. Reactive hyperaemia is another phenomenon related to local metabolic flow control mechanism. In reactive hyperaemia, brief interruption of arterial blood flow results in transient increase in blood flow that exceeds the baseline, after which the flow returns to baseline level. Both the deprivation of tissue oxygen and accumulation of vasodilating substances probably account for this phenomenon. The duration of reactive hyperaemia depends on the duration of flow cessation and usually lasts long enough to repay the oxygen debt.
Autoregulation and metabolic mechanisms control blood flow by dilation of microvasculature. The consequent increase in blood flow dilates the larger arteries upstream via the mechanism of flow-mediated dilation. The pivotal role of endothelial cells in the transduction of shear stress secondary to increased blood flow and the release of the vasodilators has been alluded to earlier. Flow-mediated dilation has been shown to occur predominantly as a result of local endothelial release of nitric oxide. 85 The mechanisms of shear stress detection and subsequent signal transduction are unclear, but probably involve opening of calcium-activated potassium channels 86–88 that hyperpolarises endothelial cells and calcium activation of endothelial nitric oxide synthase. 85,89 Flow-mediated dilation allows flow to increase with a negligible increase in pressure gradient, thus optimizing energy losses within the circulation. 90 The phenomenon of flow-mediated dilation as induced by reactive hyperaemia has commonly been used as an assessment of endothelial function in vivo. All of the aforementioned mechanisms represent relatively acute responses to regulate local blood flow. Long-term local mechanisms involve changes in tissue vascularity, release of angiogenic factors, and development of collateral circulations.
Humoral Control
Humoral control refers to regulation by hormones or locally produced vasoactive substances that act in an autocrine or a paracrine fashion. These humoral substances act either directly via receptors on vascular smooth muscle cells or indirectly through stimulation of the endothelium to release vasoactive substances.
Circulating catecholamines, noradrenaline and adrenaline, are secreted by the adrenal medulla, which is innervated by pre-ganglionic sympathetic fibres. Sympathetic activation stimulates the release of catecholamines, about 80% being noradrenaline, from the adrenal gland. The adrenal gland and the noradrenergic sympathetic vasoconstrictor fibres provide dual control of circulation by catecholamines. Adrenergic receptors in the blood vessels are α 1 , α 2 , and β 2 receptors. Noradrenaline causes vasoconstriction by acting on α-receptors, while adrenaline causes vasodilation at physiological concentrations through its β-agonistic effect. At higher concentrations, adrenaline also causes vasoconstriction through activation of α-receptors.
The regulatory role of the renin-angiotensin system in the circulation is well known. The final effector of the system, angiotensin II, mediates its effects classically in an endocrine fashion. In response to decreased renal perfusion pressure or extracellular fluid volume, renin is secreted from the juxta-glomerular apparatus of the kidney and cleaves angiotensinogen, released from the liver, to form angiotensin I. By the action of angiotensin-converting enzyme, which is predominantly expressed on the surface of endothelial cells in the pulmonary circulation, angiotension I is activated to angiotensin II. Angiotensin II is a potent vasoconstrictor and acts directly by stimulating the angiotensin II type I (AT 1 ) receptor and indirectly by increasing sympathetic tone and release of vasopressin. Recently, it has become obvious that a local paracrine renin-angiotensin system exists in the vasculature. 91,92 Vascular production of angiotensin II has been shown to be mediated by the endothelium. 93 The tissue renin-angiotensin system has dual effects on vessel function, being mediated through opposing effects of two receptors. Stimulation of AT 1 receptor causes contraction of vascular smooth muscle by directly increasing intracellular calcium and indirectly stimulating synthesis of endothelin-1 and other vasoconstrictors. 94 Furthermore, promotion of oxidative stress via the AT 1 receptor may possibly reduce nitric oxide bioavailability. 95,96 On the other hand, stimulation of angiotensin II type 2 receptor appears to mediate vasodilation through activation of the nitric oxide pathway. 97 The local tissue angiotensin II hence also plays an important role in maintaining vascular homeostasis. Additionally, recent studies have shown that other biologically active aminopeptides of the circulating renin-angiotensin system, such as angiotensins III and IV, may act in the central nervous system to raise blood pressure through the AT 1 receptor. 98
Three peptides of the natriuretic peptide family, atrial natriuretic peptide, brain natriuretic peptide, and C-type natriuretic peptide, participate in the control of circulation. The atrial natriuretic peptide is primarily produced by the atrial myocardium, while the brain natriuretic peptide is synthesised by the ventricular myocardium. The main stimulus for their release is stretching of the myocardium. Other stimuli include endogenous vasoactive factors, neurotransmitters, pro-inflammatory cytokines, and hormones. 99,100 The vascular effects of atrial and brain natriuretic peptides are similar. Both reduce sympathetic tone through suppression of sympathetic outflow from the central nervous system, reduction of release of catecholamines from autonomic nerve endings, and probably damping of baroreceptors. 101,102 The consequence is decreased vascular tone and increased venous capacitance. The atrial and brain natriuretic peptides also inhibit the activities of the renin-angiotension system, endothelins, cytokines, and vasopressin. 99,103,104 The renal haemodynamic effects include induction of diuresis, secondary to increased glomerular filtration rate due to vasodilation of afferent renal arterioles and vasoconstriction of the efferent arterioles, 105 and promotion of natriuresis. Despite preload reduction, reflex tachycardia is suppressed as these peptides lower the activation threshold of vagal afferents. C-type natriuretic peptide is a more potent dilator of veins than the other natriuretic peptides and acts in an autocrine or paracrine fashion.
Adrenomedullin was first isolated from human pheochromocytoma cells, identified by its ability to stimulate cyclic adenosine monophosphate in platelets. 106 It was subsequently found that adrenomedullin is produced in a wide range of cells, including vascular endothelial and smooth muscle cells. 107,108 Evidence is accumulating that adrenomedullin may function as a novel system in the control of circulation. 109,110 Infusion of adrenomedullin via the brachial artery in humans has demonstrated dose-dependent vasodilation and increase in blood flow. 111 Furthermore, the finding of variable blockade of the vasodilating effect of adrenomedullin by inhibition of nitric oxide synthase activity suggests that nitric oxide may be an important mediator for adrenomedullin. 112–114
The endothelium-derived vasoactive substances and their role in the control of vascular tone and homeostasis were discussed earlier. While prostaglandins are produced by most tissues, prostacyclin (prostaglandin I 2 ) is the main prostanoid produced by the endothelium of blood vessels. Prostacyclin is a potent vasodilator and an inhibitor of platelet aggregation. Nonetheless, it appears to have a limited role in humans in the control of basal vascular tone. The balance of actions between two antagonistic prostanoids, prostacyclin and thromboxane A 2 , has been the recent focus of attention in the light of reported adverse effects of selective inhibition of cyclooxgenase-2. 115,116 Prostaglandins and thromboxane are eicosanoids generated by metabolism of arachidonic acid, a major unsaturated fatty acid present in the phospholipids of cell membranes. Arachidonate is released from the membrane phospholipids by phospholipase A 2 due to a variety of mechanical and neurohumoral stimuli. Arachidonate is then converted to prostaglandin H 2 by prostaglandin H synthase, also known as cyclooxygenase. Specific synthases then produce the biologically active end products of this metabolic pathway, namely prostaglandin E 1 , prostaglandin F 2α , prostacyclin, and thromboxane A 2 . The endothelial cells produce predominantly prostacyclin and lesser amounts of prostaglandin E 1 , also a vasodilator, and prostaglandin F 2α , a vasoconstrictor. 117 Thromboxane A 2 , although predominately generated by platelets, is also synthesised by the endothelium 118 and induces vasoconstriction and platelet aggregation. Cyclooxygenase exists in two isoforms: cyclooxgenase-1 and cyclooxygenase-2. 119,120 Whether endothelial production of prostacyclin depends on cyclooxgenase-1 or -2 activity, however, remains debatable. 116,121,122 Under normal physiological conditions, eicosanoids, primarily prostacyclin, produced by the cyclooxygenase pathway induce vasorelaxation. 120 Furthermore, the cyclooxygenase-dependent vasodilators can compensate for the deficiency of other vasorelaxants. 123 By way of the lipoxygenase pathway, leucotrienes are produced from arachidonic acid. Leucotrienes C 4 , D 4 , and E 4 cause arteriolar constriction, while leucotrienes B 4 and C 4 induce pulmonary vasoconstriction by activating cyclooxygenase to produce thromboxane A 2 117
Several other endogenous substances affect the systemic circulation. Vasopressin, produced in the supraoptic and paraventricular nuclei of the hypothalamus, is probably the most potent known endogenous constrictor. It is released in quantities sufficient to exert a pressor effect when volume depletion is significant. It has, however, little role in normal vascular control. 124,125 Serotonin exists in large amount in the enterochromaffin cells of the gastrointestinal tract. Although serotonin exerts vasoconstrictor and vasodilator effects, depending on vasculature, its function in the regulation of circulation is unknown. Kinins are among the most potent endogenous vasodilators. Examples of kinins include bradykinins and kallidin, which are formed from the action of kallikrein on the α 2 -globulin kininogen. Kinins cause vasodilation and increase capillary permeability. Bradykinin is believed to play a role in the control of blood flow in the skin, gastrointestinal glands, and salivary glands. Histamine is located in mast cells and basophils. Histamine is released from these cells upon stimulation by injury, inflammation, or allergic reaction to induce vasodilation and increase capillary permeability.
Neural Control
Neural control of the systemic circulation involves feedback mechanisms that operate in both the short and long term through the autonomic, primarily the sympathetic, nervous system. 126 Short-term changes in sympathetic activity are triggered either by reflex mechanisms involving peripheral receptors or by a centrally generated response. Long-term changes, on the other hand, are evoked through modulation of the sympathetic nervous system by other humoral factors and possibly by central mechanisms involving the hypothalamus.
Peripheral receptors that constitute the afferent limb of the reflex are arterial baroreceptors, arterial chemoreceptors, and cardiac stretch receptors. Arterial baroreceptors are located in the walls of the carotid sinus and aortic arch. Afferent fibres run in the glossopharyngeal and vagal nerves and terminate within the nucleus tractus solitarius. The nucleus tractus solitarius neurons then excite neurons within the caudal and intermediate parts of the ventrolateral medulla to cause inhibition of the sympathoexcitatory neurons in the rostral ventrolateral medulla. 127 Hence, stretching of arterial baroreceptors increases afferent input and results in reflex slowing of heart rate, decrease in cardiac contractility, and vasodilation, thereby providing a negative feedback mechanism for homeostasis of arterial pressure. 128
Peripheral chemoreceptors are located in the carotid and aortic bodies, being stimulated primarily by decreased arterial partial pressure of oxygen. Their afferent fibres also run in the glossopharyngeal and vagus nerves. Activation of peripheral chemoreceptor results in hyperventilation and sympathetically mediated vasoconstriction of vascular beds, with the exception of those of the heart and brain. 129 Hence, oxygen conservation is attempted by increasing oxygen uptake and reducing tissue oxygen consumption. These chemoreflexes are nonetheless subjected to negative feedback interaction, with inhibition of the chemoreflex-mediated sympathetic activation through stimulation of baroceptors and thoracic afferents. 130
Atrial receptors are located in the walls of the right and left atriums and in pulmonary venous and cavoatrial junctions. 131,132 Two types of atrial receptors are described based on their discharge pattern in relation to atrial pressure waves. Type A receptors signal atrial contraction and hence respond to increase in central venous pressure. These receptors send impulses via myelinated fibres in the vagus nerve, while the efferent portion consists of sympathetic activation. The tachycardia due to stimulation of sinoatrial node caused by atrial stretch is termed the Bainbridge reflex. Type B baroreceptors are stretch receptors stimulated by volume distention of the atriums and fire during ventricular systole. The afferents are via unmyelinated vagal fibres. Atrial distention decreases sympathetic activity. Receptors which respond to stretch and contractility are also present in the ventricles. The receptors provide afferent input to the medulla via unmyelinated C-fibres. 133 Stimulation of these fibres decreases sympathetic tone and causes bradycardia and vasodilation. Stretching of the atrial and ventricular myocardium also leads to the release of natriuretic peptides, as discussed earlier.
Apart from the reflex-triggered short-term control of circulation, the central pathways responsible for the central command responses, such as those occurring at the onset of exercise or evoked by a threatening stimulus, are increasingly understood. 126 The rostral ventrolateral medulla is traditionally regarded as the vasomotor centre that controls circulation via the autonomic, primarily sympathetic, nervous system. 134 Nonetheless, accumulating evidence suggests that the dorsomedial hypothalamic nucleus may be a critical region responsible for the integration of autonomic, non-autonomic, and cardiovascular components of the central command responses. 135 Indeed, recent data suggests that groups of neurons in the hypothalamus can project to synapse directly with sympathetic pre-ganglionic fibres in the spinal cord, implying that the medullary vasomotor centre is perhaps not the only region that directly controls sympathetic outflow. 136
The autonomic nervous system represents the efferent component of the neural control of the circulation. Up to three types of fibres may innervate blood vessels: sympathetic vasoconstrictor fibres, sympathetic vasodilator fibres, and parasympathetic vasodilator fibres. As the size of vessel decreases, the density of autonomic innervation increases. The small arteries and arterioles are therefore the most richly innervated arteries.
Sympathetic vasoconstrictor fibres release noradrenaline upon nerve stimulation and constitute the most important components in the neural control of circulation. Post-synaptically, the α 1 -adrenoceptor is the predominant receptor mediating vasoconstriction. Although noradrenaline is the principal neurotransmitter in the sympathetic nervous system, it has been observed to co-exist with adenosine triphosphate and neuropeptide Y in sympathetic neurons. 137,138 Co-transmission refers to the concept of storage and release of more than one type of neurotransmitter, 139 which is now recognised as the norm of most neurons. Hence, in most blood vessels, adenosine triphosphate and noradrenaline act synergistically to cause vasoconstriction by acting on the post-synaptic P 2 purinoreceptors and α 1 -adrenoceptors, respectively. While neuropeptide Y has little direct action in most vessels, it appears to have an enhancing effect on the post-synaptic activity of adenosine triphosphate and noradrenaline and to act presynaptically to inhibit release of these transmitters. 140 Sympathetic vasoconstriction of arterioles increases vascular resistance, while constriction of capacitance vessels alters the circulating blood volume. In larger arteries, contraction of vascular smooth muscle in response to sympathetic activation causes less significant change in arterial caliber but alters vascular tone and hence arterial stiffness.
Sympathetic vasodilator fibres are scarce and are not tonically active. Evidence suggests that sympathetic vasodilator fibres regulate skeletal vascular tone in many animal species. Both cholinergic 141 and nitric oxide–dependent 142,143 mechanisms contribute to the vasodilator effect. Parasympathetic vasodilator fibres are found in blood vessels of the salivary gland, cerebral arteries, and coronary arteries. The vasodilator effect is mediated via release of acetylcholine with hyperpolarisation of the vascular smooth muscle.
Long-term neural regulation of the circulation is modulated by humoral and other factors. Angiotensin II is an important facilitator of sympathetic transmission. It may enhance neurotransmitter release at sympathetic nerve terminals, sympathetic transmission through sympathetic ganglia, 144 and perhaps central activation of sympathetic nervous activity. 145 Evidence is also accumulating that nitric oxide interacts with the autonomic nervous system at both central and peripheral levels. 146 Centrally, nitric oxide decreases sympathetic vasoconstrictor outflow. Peripherally, augmented vasoconstriction to nitric oxide synthase inhibition has been demonstrated in denervated forearm in humans. 147 Interaction between nitric oxide and cholinergic vasodilator fibres is also evidenced by significant pressor response to nitric oxide synthase inhibition with cholinergic blockade. 148 Finally, accumulating evidence suggests that the hypothalamic paraventricular nucleus in the central pathways may mediate sustained increases in sympathetic nerve secondary to a variety of stimuli. 127 It has therefore been hypothesised that stress, anxiety, or pathological conditions such as heart failure may exert long-term influence on neural control of circulation through tonic activation of sympathoexcitatory neurons located in paraventricular nuclei of the hypothalamus. 126
MODELING OF SYSTEMIC CIRCULATION
Models
From the mechanical perspective, the systemic arterial system can be envisaged as a network of elastic tubes that receive pulsatile blood flow from left ventricular ejection and transmit it distally as a steady stream into capillaries. Hence, apart from acting as a low-resistance conduit, the systemic arterial tree functions as a cushion to smooth out pressure and flow pulsations generated by cycles of left ventricular contraction. While the success of the conduit function depends primarily on a low peripheral vascular resistance, the efficiency of cushioning function depends on the elastic properties, described in terms of stiffness, of the arterial system. Importantly, stiffness of the arterial system is related to vascular impedance, the opposition to blood flow taken to represent the afterload presented by the systemic arterial circulation to the left ventricle.
Modeling of the arterial circulation has contributed significantly to the understanding of the behaviour of the arterial system and the effects of arterial load on the systemic ventricle. The lumped model of arterial circulation, commonly termed Windkessel model, was first described in the eighteenth century. In his book entitled Haemastaticks, Hales drew an analogy between the arterial system and an air-filled dome of the fire engine compression chamber ( Windkessel ) 149 ( Fig. 6-1 ). The dome represents the cushioning function of the arteries, which buffers intermittent spurts of water from the pump. The rigid fire hose represents the conduit function, while the nozzle represents the peripheral resistance. As an analogy, blood ejected from the left ventricle distends the large elastic arteries during systole, while elastic recoil of the arteries during diastole propels blood to perfuse the peripheral resistance vessels. This cushioning function smooths out the pulsatile blood flow and protects the peripheral vascular beds from exposure to large pressure fluctuations. The electrical analogues of the systemic arterial system are shown in Figure 6-2 . The two-element electrical analogue of the Windkessel model comprises a capacitor, which represents the arterial compliance, and a resistor, the total peripheral resistance. To better characterise the arterial system, a modified Windkessel model has been proposed 150 to take into account the input impedance of the proximal aorta by addition of a resistor proximal to the two-element capacitance-resistance model. A four-element Windkessel model, with addition of an inertial term, has further been shown to be superior to the three-element Windkessel as a lump model of the entire systemic tree. 151 Inertance is due to mass of the fluid and physiologically; it can be regarded as the inertial effect secondary to simultaneous acceleration of the blood mass within the vessel.


The Windkessel model, albeit simple and qualitative, emphasises the elasticity of the large arteries and resistance offered by the small peripheral arteries. Nonetheless, this model has intrinsic shortcomings: limitation of vessel elasticity to one site, lack of a finite velocity of propagation of the pulse wave, and overlooking of the significance of wave reflection. In this model, the pressure generated by contraction of the systemic ventricle is assumed to be transmitted instantaneously throughout the windkessel , the pressure pulse is assumed to subside before the next cardiac cycle, and there is a single systolic and a single diastolic blood pressure throughout the major arteries. However, the fact that the cushioning and conduit functions of the arterial tree are combined results in two phenomena: (1) traveling of pulse wave at a finite speed along the arterial wall, and (2) wave reflection at arterial terminations and other discontinuities. A more realistic model proposed is a distensible tube with one end receiving pulsatile ejection of blood from the left ventricle and with the other end representing the peripheral resistance. 152 The pressure wave at any point along the tube is regarded as a result of the incident and reflected waves. The velocity at which the pulse travels depends on the elasticity of the tube and has implications on the timing of arrival of the reflected wave. When the tube is distensible, the wave velocity is slow and the reflected wave returns late in diastole. By contrast, when the tube is stiffened, the pulse velocity is increased and the reflected wave merges with the systolic part of the incident wave, causing a higher pressure in systole and a lower pressure in diastole. It is conceivable, therefore, that stiffness is an important mechanical property of the arterial tree and contributes to left ventricular afterload.
Arterial Impedance as Ventricular Afterload
Ventricular afterload can be conceptualised as all those external factors that oppose ventricular ejection and contribute to myocardial wall stress during systole. The hydraulic load of the systemic arterial system has therefore been taken to represent the afterload presented to the systemic ventricle. 153,154 The total arterial hydraulic load comprises three components: resistance, stiffness, and wave reflection, all of which can be obtained from impedance spectra based on analysis in the frequency domain. 155
Vascular Resistance
Vascular resistance is commonly used in the clinical setting as an index of systemic ventricular afterload. The electrical analogue for vascular resistance is described by Ohm’s law, which applies to direct electric current circuit. For a steady flow state, the vascular resistance is derived by dividing pressure gradient by volume flow. As the systemic venous pressure is very small when compared with the mean aortic pressure, the systemic arterial resistance can be approximated as mean aortic pressure divided by cardiac output. Nonetheless, as arterial blood flow is pulsatile in nature, the use of vascular resistance alone to describe afterload is deemed inadequate.
Vascular Impedance
For pulsatile flow, the corresponding pressure-flow relationship is vascular impedance. This is analogous to the voltage-current relationship of an alternating current electric circuit. To analyse the mathematical relationship between pressure and flow waves, Fourier analysis is used to decompose these complex non-sinusoidal waves into a set of sinusoidal waves with harmonic frequencies that are integral multiples of the fundamental wave frequency ( Fig. 6-3 ). Each of the sinusoidal components is described in terms of frequency, amplitude, and phase angle.

In applying Fourier analysis to the circulatory system, two prerequisites have to be fulfilled: periodicity and linearity. Regularity of the heartbeat can generally be regarded as a type of steady-state oscillation. 156 For a linear system, when the system is driven by a pure sine wave of a certain frequency, no pressure or flow components of another frequency should be generated. By contrast, there will be interactions between harmonic components and creation of additional frequency components in a non-linear system. Hence, strictly speaking, the harmonic terms of the pressure should be related exclusively to the corresponding harmonic terms of flow if the circulatory system is linear. While non-linearities of the circulatory system do exist, their magnitude is small. 157 Importantly, the arterial system has been shown to be linear for normal physiological oscillations. 156,158
Vascular input impedance is defined as the ratio of pulsatile pressure to pulsatile flow. The aortic input impedance is particularly relevant as it characterises the properties of the entire systemic arterial circulation and represents the hydraulic load presented by the systemic circulation to the left ventricle. 153,154 To obtain the aortic input impedance spectrum, the ascending aortic flow is usually measured by an electromagnetic flow catheter, while the pressure is measured by a micromanometer mounted onto the catheter. Noninvasive determination of aortic input impedance involves the use of Doppler echocardiography to measure flow and tonometry to obtain a carotid, subclavian, or synthesised aortic pressure waveform, the latter based on the radial arterial waveform.
An example of the human aortic input impedance spectra is shown in Figure 6-4 . The vascular impedance modulus at different harmonics is the ratio of pressure amplitude to flow amplitude. For a heart rate of 60 beats/min, the fundamental frequency is 1 Hz, the second harmonic is 2 Hz, and so forth. Beyond the 10th harmonic, the magnitudes are usually small and negligible. At zero frequency, impedance is equivalent to resistance in the steady-flow state. The phase difference is the delay in phase angle between the pressure and flow harmonics, which is analogous to time delay in the time domain. When a particular pressure harmonic leads the flow harmonic, the phase angle is positive. Conversely, when the pressure harmonic lags behind the corresponding flow harmonic, the phase is negative. The aortic input impedance spectrum is characterised by a steep decrease in the magnitude of input impedance from its value at zero frequency (resistance), followed by fluctuations with maxima and minima. The fluctuations of the impedance modulus are related to peripheral wave reflection as discussed in the following section. The average of the relatively stable high-frequency components of the impedance moduli provides an approximation of characteristic impedance.

Characteristic impedance is the ratio of pulsatile pressure to pulsatile flow at a site where pressure and flow waves are not influenced by wave reflection. The concept of characteristic impedance is important as it is principally determined by and related directly to stiffness of the major arteries distal to the site of measurement. Hence, it represents the pulsatile component of the hydraulic workload presented to the left ventricle when it is measured at the ascending aorta. As wave reflection is always present, characteristic impedance cannot be measured directly. It is usually estimated by averaging impedance moduli over a frequency range where fluctuations due to wave reflection above characteristic impedance are expected to cancel out those below. 159 Hence, characteristic impedance has been estimated as the average value of modulus between 2 and 12 Hz, 160 above 2 Hz, 161 or above the frequency of the first minimum. 152 In the time domain, the characteristic impedance can be estimated by relating the initial upstroke of the pressure wave to the upstroke of the simultaneously recorded flow wave, 162 as the effects of wave reflection are minimal with the first 20 msec of the wave.
Wave Reflection
As the velocities of pressure and flow waves transmitted in the arteries are of the order of metres per second, it is obvious that the waves have sufficient time to travel to the periphery and be reflected back before the next cardiac cycle. The existence of wave reflections is supported by several observations. A secondary pressure wave is usually obvious in arterial pressure pulse when flow is in fact decreasing. Furthermore, such secondary pressure waves show different patterns in different arteries. 163,164 Wave reflection also accounts for the observed amplification of the pulse between central and peripheral arteries. 152
As a result of non-uniformities of geometric and elastic properties along the arterial tree and impedance mismatch at the arterial termination, wave reflection sites can exist throughout the arterial system. There is no universal agreement on the reflecting sites, with possible ones including branching points in major arteries, 165,166 areas of alterations in arterial stiffness, 167 and high-resistance arterioles. 152 Nonetheless, the terminations at which low-resistance conduit arteries terminate in high-resistance arterioles are usually regarded as the principal sites for reflection. Wave reflection in the ascending aorta hence represents the result of reflections at multiple peripheral sites of the body.
The pressure and flow waves measured at any site in the arterial system can therefore be considered as a summation of a forward or incident wave and a reflected wave. Wave reflection exerts opposite effects on pressure and flow. Reflected pressure wave increases the amplitude of the incident pressure wave, while reflected flow wave decreases the amplitude of the incident flow wave. In most experimental animals and in young human subjects who have elastic arteries, wave reflection returns to the ascending aorta from the periphery after ventricular ejection. 152 Such timing is desirable, as the reflected pressure wave augments early diastolic blood pressure, thereby boosting the perfusion pressure of the coronary arteries without increasing left ventricular afterload.
Alteration of arterial stiffness has profound effects on wave reflection. Stiffening of systemic arteries due to aging or disease processes increases pulse wave velocity and causes earlier return of the reflected wave to augment aortic blood pressure in late systole rather than in diastole, the implications of which will be discussed in the section on ventriculo-arterial interaction.
MEASURES OF ARTERIAL FUNCTION
Arterial Stiffness
Arterial stiffness describes the rigidity of the arterial wall. In the last decade, there has been increasing interest in the potential role of arterial stiffening in the development of cardiovascular disease in adults. Arterial stiffness is primarily determined by structural components of the arterial wall, elastin and collagen in particular, vascular smooth muscle tone, and transmural distending pressure. 168 Increasing evidence suggests a role for endothelium in the regulation of arterial stiffness through the influence of smooth muscle tone by release of vasoactive mediators. Indeed, the influence of basal nitric oxide production 169 and endothelin-1 170 on stiffness of the common iliac artery in an ovine model have recently been shown. Additionally, it has been shown that atrial natriuretic peptide and, to a lesser extent, brain natriuretic peptide can modify iliac artery stiffness in this animal model. 171
The significance of arterial stiffness, as alluded to earlier, owes to its direct relationship with characteristic impedance, hence the pulsatile component of the arterial afterload, and its effect on the timing of return of the reflected waves from peripheral sites. On the one hand, atherosclerotic changes with thickening, fibrosis, and fragmentation and loss of elastin fibres can stiffen the arterial wall by causing structural alterations 172 ; on the other, arterial stiffening may predispose the intima to atherosclerosis due to injury sustained from increased pulsatile pressure. 173 Indeed, aortic stiffness has been positively associated with the extent of coronary arterial plaque load in elderly subjects undergoing elective coronary angiography. 174
The contention that arterial stiffness is a marker of vascular disease and a risk factor for cardiovascular morbidity and mortality in adults is gaining support, and the role of arterial stiffness in the development of cardiovascular disease is increasingly emphasised. 175 The association in adults of increased arterial stiffness and various pathophysiological conditions, which are themselves also associated with increased cardiovascular risk, has been extensively reviewed. 175–178 Studies have also shown that arterial stiffness is associated with end-organ alterations including left ventricular hypertrophy and arterial intima-media thickening in adults independent of systemic blood pressure. 179 Importantly, stiffness of central arteries, as assessed by aortic pulse wave velocity and carotid distensibility, has been shown to have independent predictive value for cardiovascular events in the general adult population, 180,181 in the elderly, 182 in adults with hypertension, 183–185 in end-stage renal failure, 186–189 and with impaired glucose tolerance. 190
While central arterial stiffness has been the focus of most of the adult studies, the contribution of stiffness of the smaller peripheral arteries to total vascular impedance should not be ignored. Structural remodeling occurs also in smaller arteries and branching points. The changes in mechanical properties of conduit and resistive arteries influence wave reflections and contribute to augmentation of late systolic blood pressure in the aortic root. 191 Hence, carotid augmentation index has also been shown to have independent predictive value for cardiovascular events in adults with hypertension, 192 and end-stage renal failure 193 and in those undergoing percutaneous coronary interventions. 194 Associations between increased small artery stiffness, as assessed by pulse contour analysis, and aging, hypertension, smoking, diabetes, and cardiovascular events have also been reported. 195,196
The increasing application of non-invasive methods to determine systemic arterial stiffness in the clinical and research arenas has significantly increased the understanding of its pathophysiological significance. With adoption of these non-invasive methodologies for use in children and adolescents, 197,198 the phenomenon and significance of arterial stiffening in the young is also beginning to unveil.
Measurement of Arterial Stiffness in Vivo
Non-invasive methods for determination of local, regional, and systemic arterial stiffness and quantification of wave reflections in vivo are available. For meaningful interpretation of these indices, their fundamental limitations have to be taken into account. First, the relationship between pressure and arterial diameter is nonlinear due to progressive recruitment of the stiffer collagen as transmural pressure increases. Arterial stiffness should therefore be quantified at a given level of pressure as the tangent to the pressure-diameter curve. 152 Importantly, comparison of arterial stiffness among different populations should take into account the potential confounding influence of the distending pressure. Second, modulation of smooth muscle tone by sympathetic nervous activity, hormones, or endothelium-derived vasoactive substances as mentioned above can alter arterial stiffness. Finally, spontaneous vasomotor changes in the muscular arteries can alter arterial diameter and stiffness. 199
Local Arterial Stiffness
Local arterial stiffness is obtained by relating pressure changes to arterial diameter or cross sectional area changes at the site of interest. Arterial stiffness can be expressed as compliance, distensibility, Person’s elastic modulus, Young’s modulus, and stiffness index 175,200,201 ( Table 6-1 ). The elastic property of the artery as a hollow, circular structure is described by distensibility, compliance, Peterson’s elastic modulus, and stiffness index. The elastic property of the arterial wall, on the other hand, is estimated by Young’s elastic modulus that takes into account the wall thickness, which is usually estimated by the intima-media thickness. Assumption of homogeneity of the non-homogeneous arterial wall, however, underestimates Young’s elastic modulus. Among the various indices of local arterial stiffness, the stiffness index is considered relatively independent of systemic blood pressure. 202
Term | Definition | FORMULA | |
---|---|---|---|
in Terms of Change in Vessel Diameter | in Terms of Change in Cross Sectional Area of Vessel Lumen | ||
Compliance | Absolute change in diameter or area during systole for a given pressure change | ΔD/ΔP | ΔA/ΔP |
Distensibility | Relative change in diameter or area during systole for a given pressure change | ΔD/(D · ΔP) | ΔA/(A · ΔP) |
Peterson’s elastic modulus | Inverse of distensibility, i.e., the pressure change required for a given relative change in diameter or area | ΔP · D/ΔD | ΔP · A/ΔA |
Stiffness index (β) | Ratio of ln (systolic/diastolic pressure) to relative change to vessel diameter | ln (Ps/Pd)/(ΔD/D) | |
Young’s modulus or incremental elastic modulus | Elastic modulus per unit wall thickness or area; provides information on intrinsic elastic properties of the arterial wall | ΔP · D/ΔD · h |
|
For superficial arteries such as the brachial, femoral, and carotid arteries, the diameter and diameter change from end-diastole to systole can be assessed by ultrasound and echo-tracking techniques. Two-dimensional ultrasound assessment is, however, limited by the precision of measurements. In contrast, echo-tracking devices process radiofrequency signals to track the displacement of the anterior and posterior arterial walls with a high precision. 203,204 The difference between displacements, which reflects changes in arterial diameter as a function of time, can then be displayed ( Fig. 6-5 ). The precision in determining the change in diameter has been estimated to be as low as 1 μm for echo-tracking devices and about 150 μm for video-image analysis of ultrasound images. 175,203,205 Furthermore, the intima-media thickness can be estimated from the radio-frequency signals, which enable calculation of Young’s elastic modulus. An additional advantage of echo-tracking devices is that a pressure-diameter curve can be plotted for determination of arterial stiffness at any given blood pressure. 168,206 For deeper arteries such as the aorta, cine magnetic resonance imaging 207 and transoesophageal echocardiography with acoustic quantification 208 have been used to determine diameter change during the cardiac cycle.

Ideally, the local pressure should be measured at the site of diameter measurements. Applanation tonometry allows noninvasive recording of the arterial pressure waveform in the carotid and peripheral conduit arteries. 209 Gentle compression of the superficial artery against the underlying bone by the pen-like tonometer allows its equalisation with the arterial circumferential pressure. The recorded pressure waveform, almost identical to that obtained intra-arterially, can then be calibrated against the cuff mean and diastolic blood pressures of the brachial artery. 210,211 Derivation of central aortic waveform from radial arterial tonometry has also been made possible by application of a transfer function, which has been validated in adults 212–214 but not in children. Although cuff brachial artery pulse pressure has commonly been used for the calculation of local arterial stiffness indices, amplification of pressure pulse along the arterial tree constitutes a potential source of error.
Regional Arterial Stiffness
Arterial stiffness of an arterial segment, or regional stiffness, is assessed by measuring the pulse wave velocity over the segment of interest. Pulse wave velocity is the speed at which the pressure or flow wave is transmitted along the arterial segment. It is related to Young’s elastic modulus ( E ) by the Moens-Korteweg equation: PWV = √ E h/2rρ, where PWV is pulse wave velocity, h is wall thickness of vessel, r is inside radius of vessel and ρ is density of blood. 152 The Bramwell and Hill 215 (1922) equation relates pulse wave velocity to arterial distensibility: PWV = √(ΔP·V)/ΔVρ = √1/ρD, where P is pressure, V is volume, ΔP·V/ΔV represents volume elasticity, and D is volume distensibility of the arterial segment. Furthermore, pulse wave velocity is directly related to characteristic impedance (Z c ) by the formula 166 Z c = PWV·ρ. Pulse wave velocity is hence related directly to arterial elasticity and characteristic impedance and inversely to arterial distensibility. By providing an average stiffness of the arterial segment of interest, pulse wave velocity may provide a better reflection of the general vascular health. As aforementioned, the value of aortic pulse wave velocity as a risk for cardiovascular morbidity and mortality in adults is increasingly recognised. 175
Pulse wave velocity is determined by dividing the distance of pulse travel by the transit time. As the pressure pulse and flow pulse propagate at the same velocity, the arterial pulse may be registered using pressure-sensitive transducers, 216 oscillometric devices, 217 applanation tonometry, 218 and Doppler ultrasound. 219,220 Furthermore, the pulse wave can be detected using magnetic resonance imaging, 221,222 which also allows accurate determination of path length and measurements to be made from relatively inaccessible arteries. Recently, determination of pulse wave velocity based on the principle of photoplethysmography 223 has also been validated. 224 By contrast to the assessment of local arterial stiffness, measurement of pulse wave velocity does not require accurate measurement of the pressure in the arterial segment of interest.
Transit time is measured as the time delay between the feet of the proximal and distal pulse waves ( Fig. 6-6 ). The time delay can be measured by simultaneously recording the pulse waves at two sites of the arterial segment. Alternatively, the time intervals between the R-wave of the electrocardiogram and the foot of the pulse wave at the two sites may be recorded consecutively, and the transit time calculated as the difference between the two. The foot of the pulse wave is used to locate the wave front as it is relatively unaffected by wave reflections. The most consistent method for determination of the foot of the pulse wave has been shown to be either the point at which its second derivative is maximal or the point formed by intersection of a line tangential to the initial systolic upstroke of the waveform and a horizontal line through the minimum point. 225

The distance can be estimated by direct superficial measurement between the centres of the two pressure transducers or other devices in case of relatively straight segments like the brachioradial arterial segment. If arterial segments are not straight, measurement of the distance may be a source of error. Furthermore, in two sites where pulse waves propagate in opposite directions, as in the determination of carotid-femoral pulse wave velocity, the method of measuring distance varies. Some investigators recommend using the total distance between the carotid and femoral sites of measurement, while others subtract the carotid-sternal notch distance from the total distance, or subtract the carotid-sternal notch distance from the femoral-sternal notch distance. 175,226,227 Despite the limitations of the need to estimate distance by superficial measurement, pulse wave velocity is probably the most widely used technique for assessment of arterial stiffness.
Systemic Arterial Stiffness
Pulse contour analysis has been used to assess systemic or whole-body arterial stiffness non-invasively. 228–230 One of the methods, based on the electrical analogue of a modified Windkessel model with proximal and distal capacitance, inertance, and resistance parameters, concentrates on analysis of the diastolic pressure decay of the radial pulse contour. An algorithm is used to determine the best set of values for matching the diastolic portion of the pulse contour to a multi-exponential waveform equation. Based on these values, the capacitative compliance of the proximal major arteries and the oscillatory compliance of the distal small arteries are estimated. However, the biologic relevance of the lumped proximal and distal compliance derived from a model construct based on assumptions remains to be defined. Nonetheless, these parameters have been shown to change with aging and in diseases associated with increased cardiovascular events, 228,229 although evidence for their predictive value for the occurrence of such events is lacking.
The area method, based on a two-element Windkessel model, has also been used to determine systemic arterial compliance using the formula compliance = Ad/[TVR × (Pes − Pd)], where Ad is area under the diastolic portion of the arterial pressure wave from end-systole to end-diastole, TVR is total vascular resistance, Pes is end-systolic pressure, and Pd is end-diastolic pressure. 231,232 The pressure and pressure waveform can be obtained by applanation tonometry over the right common carotid artery, while total vascular resistance is calculated as mean blood pressure divided by mean aortic blood flow, the latter obtained by a velocimeter positioned at the suprasternal notch. The area method nonetheless shares similar limitations.
Wave Reflection Indices
Arterial stiffening increases pulse wave velocity and shortens the time for the pulse wave to return from the periphery. Early arrival of the reflected waves augments systolic blood pressure in stiff arteries. The effects of wave reflection can be quantified by determination of this pressure wave augmentation. 166,233 The augmentation index is defined as the ratio of difference between systolic peak and inflection point to pulse pressure ( Fig. 6-7 ). The inflection point corresponds to the time when peak blood flow occurs in the artery. In adolescents and young adults with elastic arteries, the augmentation index is negative, as late return of reflected waves during diastole causes the peak systolic pressure to precede an inflection point. By contrast, in middle-aged and older individuals, the peak systolic pressure occurs in late systole after an inflection point and the augmentation index becomes increasingly positive with age. The aortic augmentation index is hence negative in adolescents and reaches to about 50% of pulse pressure at 80 years. 152 As height is related to reflection sites, the augmentation index is inversely related to height 234 and is paradoxically greater in infants and young children than in adolescents due to early return of reflected wave with a short body length. Rather than a direct measurement of arterial stiffness, augmentation index is a manifestation of arterial stiffness. It is also important to recognise that apart from arterial stiffness, the amplitude of reflected wave, reflectance point, heart rate, and ventricular contractility are all important determinants of augmentation index.

To determine the aortic augmentation index non-invasively, the central aortic waveform can be estimated from the common carotid artery waveform using applanation tonometry, although this is technically demanding. Alternatively, the aortic waveform can be reconstructed using a transfer function from the radial waveform, which is easier to obtain. 212–214 The radial-to-aortic transfer function has nonetheless not been validated in children, and furthermore, its accuracy for derivation of aortic augmentation index has been disputed. 235–237 The recent introduction of a carotid sensor with multiple micro-piezo-resistive transducers may facilitate the derivation of augmentation index. 217
Contour analysis of the digital volume pulse has also been used to derive indices attributable to wave reflection. 238–240 Takazawa et al used the second derivative of the digital photoplethysmogram waveform to identify five distinct waves ( Fig. 6-8 ) and determined their mathematical relationships. In particular, the d/a and b/a ratios have been related to age and arterial stiffness. 238,241–243 The d/a ratio is also related to aortic augmentation index. 238 However, the physical and physiological meanings of these measurements remain unclear.

Using similarly photoplethysmographic digital volume pulse waveform, Chowienczyk et al proposed that the first peak in the waveform corresponds to a forward-traveling pressure wave from the heart to the finger, and the second peak or point of inflection to the backward-traveling reflected pressure 239,240 ( Fig. 6-9 ). A reflection index, defined at the ratio of the magnitude of the reflected wave to the first peak, has been proposed as measure of the amount of reflection, while the peak-to-peak time has been proposed as a surrogate measure of pulse wave velocity and arterial stiffness. 239,240 An index of large arterial stiffness, defined as height divided by peak-to-peak time, has been shown to be related to pulse wave velocity. 244 The simplicity of the digital photoplethysmographic waveform analysis may facilitate large-scale epidemiological studies. These indices, nonetheless, provide at best an indirect assessment of arterial stiffness, and factors affecting their reliability and interpretation in different patient cohorts remain to be clarified.

Blood Pressure Indices
Central pulse pressure, being influenced by wave reflection amongst other factors, is often considered a surrogate of arterial stiffness. 245 It should not, however, be used interchangeably as an index of arterial stiffness as their physiological meanings differ. Similar to augmentation index, central pulse pressure is dependent on heart rate, ventricular contractility, and factors affecting the reflected wave, notably arterial stiffness and reflectance points. 175 Peripheral pulse pressure, measured usually at the brachial artery, overestimates central pulse pressure due to the amplification phenomenon, which is more prominent in more elastic arteries such as those in young subjects. 246
Recently, an ambulatory arterial stiffness index has been proposed as a novel index of arterial stiffness. 247,248 Using ambulatory blood pressure monitoring data throughout the day, the index is calculated as 1 minus the regression slope of diastolic blood pressure on systolic blood pressure. This index is based on the concept that average distending pressure varies during the day and that the relation between diastolic and systolic blood pressure, with this changing distending pressure, largely depends on the structural and functional characteristics of the large arteries. 248 While this index has been shown to be related to pulse pressure and augmentation index and a predictor of cardiovascular mortality in adults, 247,248 its physiological meanings and its use as a marker of stiffness remain highly disputed. 175,249–251
Endothelial Dysfunction
Endothelial dysfunction is characterised by upset of the regulation of balance between vasodilation and vasoconstriction, inhibition and promotion of vascular smooth muscle proliferation, and prevention and stimulation of platelet aggregation, thrombogenesis, and fibrolysis by the endothelium. 252 While the normal quiescent state is represented by nitric oxide–dominated endothelial phenotype and maintained primarily by laminar shear stress, 253 endothelial activation is characterised by dominance of reactive oxygen signaling. 254 The common denominator of chronic production of reactive oxygen species potentially exhausts the protective capacity of endogenous anti-inflammatory and anti-oxidative mechanisms and results in sustained endothelial dysfunction. Dysfunction of the endothelium results in loss of its protective function, increased expression of adhesion molecules, and promotion of inflammation within the vessel wall.
Given the important protective role of the endothelium against vascular injury, inflammation, and thrombosis, all of which are key events involved in the initiation and progression of atherosclerosis, it is not surprising that endothelial dysfunction has also prognostic implications. In adults with or without coronary atherosclerosis and in those with hypertension, coronary endothelial dysfunction, 255–258 impaired flow-mediated dilation of the brachial artery, 259–261 and impaired agonist-mediated increase in forearm blood flow 262,263 have been shown to predict cardiovascular events. With the introduction of a variety of non-invasive techniques as elaborated below for assessment of endothelial function, the phenomenon of endothelial dysfunction has also been documented in an increasing number of paediatric and adolescent conditions.
Assessment of Endothelial Function in Vivo
Coronary Circulation
Assessment of endothelial function in the coronary circulation was first described in 1986 by Ludmer and colleagues 264 who demonstrated that local infusion of acetylcholine dilates angiographically normal epicardial coronary arteries secondary to release of nitric oxide from an intact endothelium. By contrast, acetylcholine was found to cause paradoxical constriction of atherosclerotic coronary arteries as a result of direct muscarinic action on vascular smooth muscle. Endothelial function of the coronary resistance vessels can be assessed simultaneously using Doppler flow wires. 265 The measurement of changes in coronary arterial diameter, blood flow, and vascular resistance in response to intracoronary infusion of acetylcholine has become the gold standard against which other tests of endothelial function have been compared. Endothelial-independent changes in coronary diameter and flow reserve can be assessed by intracoronary boluses of adenosine or infusion of nitroglycerine. The response to endothelium-independent agonist is assessed to exclude insensitivity of vascular smooth muscle to nitric oxide. In children, coronary endothelial function has been mainly assessed in those with a history of Kawasaki disease. The invasive nature of this technique limits its use to patients in whom cardiac catheterisation is clinically indicated and precludes serial follow-up assessments.
Forearm Resistance Vessels
Endothelial function of forearm resistance vessels is assessed by measurement of forearm blood flow in response to intra-arterial infusion of endothelium-dependent and-independent agonists. Venous occlusion plethysmography has been widely used to measure forearm blood flow. 266 The principle of measurement is based on the premise that interruption of venous outflow from the forearm, but not arterial inflow, results in a linear increase in forearm volume with time. In this technique, blood pressure cuffs are placed around the upper arm and the wrist. The forearm is positioned above the heart level to ensure satisfactory venous emptying when cuffs in the upper arm are deflated. The contralateral arm is studied simultaneously to correct for possible changes in basal blood flow with time and to act as control. The upper arm cuff is inflated to around 40 mmHg to occlude venous outflow while allowing arterial inflow into the forearm. The hands are excluded from the circulation by inflating the wrist cuff to supra-systolic blood pressure, as they contain a high proportion of arterio-venous shunts and their blood flow is highly temperature sensitive. The change in forearm volume with continuous arterial inflow is measured using strain-gauge plethysmography. The mercury-in-rubber strain gauges, which act as resistors connected as one arm of a Wheatstone bridge, 267 are placed around the right and left forearms. With the increase in forearm volume and circumference, the strain gauges lengthen and increase in resistance, leading to a potential difference in the Wheatstone bridge circuit. The period of measurement, during which the hands are rendered ischaemic, has been up to 13 minutes in adults. 268
A variety of agonists, which include acetylcholine, methacholine, bradykinin, 5-hydroxytryptamine, and substance P, have been used to assess the endothelial-dependent vasodilation response. 269,270 Of importance to note is that nitric oxide dependence varies among agonists, and indeed, vasodilation caused by methacholine does not appear to be nitric oxide mediated. 271,272 Apart from stimulation of nitric oxide release, these agonists also induce release of endothelium-derived hyperpolarizing factor and prostaglandins. The response to endothelium-independent agonist, like sodium nitroprusside, is assessed to exclude abnormal vascular smooth muscle function. The forearm blood flow response to endothelial-dependent agonist has been found to correlate with coronary endothelial function 272,273 and to predict independently cardiovascular events in patients with coronary artery disease. 262 The basal release of nitric oxide in the basal forearm can also be assessed using this technique by infusing NG-monomethyl- l -arginine, an l -arginine analogue that inhibits nitric oxide synthase. 274 Co-infusion of NG-monomethyl- l -arginine with specific endothelium-dependent agonist can further be used to determine upregulation of alternative vasodilator pathways in condition of endothelial dysfunction. 269 This technique is hence invaluable in elucidating mechanisms that underlie endothelial dysfunction. However, as a technique to assess endothelial function, the need for arterial cannulation limits its repeatability and its application in the paediatric population.
Conduit Artery
Non-invasive assessment of flow-mediated dilation of the brachial artery using high-resolution ultrasound was first introduced by Celermajer et al 275 in 1992, based on the principle of endothelium-dependent release of nitric oxide in response to shear stress. As alluded to earlier, flow-mediated dilation has been shown to occur predominantly as a result of local endothelial release of nitric oxide. 85
In this technique, the brachial arterial diameter and Doppler-derived flow velocity is determined at baseline, when the patient has rested in a supine position for at least 10 minutes, and after an increase in shear stress induced by reactive hyperaemia. To induce reactive hyperaemia, a sphygmomanometer cuff, placed either above the antecubital fossa or over the forearm, is inflated to supra-systolic blood pressure and deflated after 4 to 5 minutes. Cuff occlusion of the upper arm has additional direct ischaemic effect on the brachial artery. There is no consensus, however, as to whether cuff occlusion of the upper arm or forearm provides more accurate information. 276,277 Reactive hyperemia after cuff deflation increases shear stress and leads to dilation of the brachial artery. The maximum increase in flow is assessed within the first 10 to 15 seconds after cuff release, while the brachial arterial diameter is measured at usually 60 seconds after cuff deflation, at a time when maximal dilator response occurs in normal subjects. 278 Commercially available automatic edge-detection software allows continuous monitoring of the brachial arterial diameter after cuff deflation and detection of the true peak response ( Fig. 6-10 ). Flow-mediated dilation in the radial, femoral, and posterior tibial arteries can similarly be determined by inflating the cuff at the wrist, just beneath the popliteal fossa, and the ankle, respectively. After at least 10 minutes following cuff release, sublingual nitroglycerine is given to assess endothelium-independent vasodilation. This direct vasodilation response peaks at 3 to 5 minutes after administration of nitroglycerine.

Accurate assessment of flow-mediated dilation is nonetheless challenging. Furthermore, centres differ in the protocols used for elicitation of reactive hyperaemia. The methodological issues, strengths, and limitations of this technique have been explored in depth by the International Brachial Artery Reactivity Task Force 279 and the Working Group on Endothelin and Endothelial Factors of the European Society of Hypertension. 270 Notwithstanding the skills required and limitations of this technique, its non-invasive nature and its correlation with coronary endothelial function 280 have led to its widespread use in clinical trials and in the field of vascular epidemiology. 281–283 The technique is also widely used for the assessment of endothelial function in children and adolescents, although its application in children younger than 6 years is likely to be difficult given the cooperation needed for accurate measurement of brachial arterial diameter and flow.
Microvasculature
Laser Doppler techniques have been used for the assessment of microvascular endothelial function of the skin. 284 The principle of laser Doppler techniques is based on changes in wavelength of the reflected light after hitting moving blood cells. Rather than measuring absolute skin perfusion, these techniques determine red blood cell flux as defined by the product of velocity and concentration of the moving blood cells within the measured volume. Laser Doppler flowmetry is a single-probe technique used to assess the blood flux in a small volume of 1 mm 3 or smaller, 285 while laser Doppler imaging provides an integrated index over a larger volume by using a mirror to reflect the laser beam to scan a larger skin area. 286
To assess microvascular endothelial function, laser Doppler flowmetry and imaging have been used to detect the increase in skin blood flow during reactive hyperaemia after brief arterial occlusion, 287,288 during local thermal hyperaemia, 289,290 and after local application of endothelial-dependent vasodilators by iontophoresis. 291,292 Nonetheless, the microvascular response to reactive hyperaemia and local heating is complex and depends on mechanisms other than those mediated by the endothelium. 293–296 Iontophoresis of acetylcholine and sodium nitroprusside has commonly been used to generate endothelium-dependent and endothelium-independent dilation respectively of the skin microvasculature. The applied current can, however, induce vasodilation directly, which is more pronounced with cathodal delivery of acetylcholine and related probably to induction of an axon reflex. 297 This effect can be reduced by the use of lower anodal current and larger iontophoresis electrodes. The usefulness of acetylcholine iontophoresis as an assessment of skin microvascular endothelial function has recently been challenged, as acetylcholine-induced vasodilation has been shown to remain unchanged or be only partially attenuated after inhibition of nitric oxide synthase. 298 While the test is non-invasive and may reflect to a certain extent the microvascular endothelial function, the poor reproducibility remains an issue of concern. Despite the limitations, acetylcholine iontophoresis coupled with laser Doppler imaging have been used to assess microvascular endothelial function in neonates. 299 The latest development of laser flowmetry is quantification of periodic oscillations of skin blood flow by spectral analysis of laser Doppler signals. 300,301 The oscillations at around 0.01 Hz have been suggested to be endothelium-dependent, although further studies are required to clarify its usefulness as a test for skin microvascular endothelial function.
Emerging Techniques
The aforementioned techniques primarily assess the endothelial-dependent nitric oxide–mediated vasodilation response to agonists. Recent techniques attempted to determine the change in arterial stiffness upon endothelial stimulation. Salbutamol, a β-2 agonist, has been demonstrated to reduce arterial stiffness in a nitric oxide–dependent manner. 302 Thus, the changes in augmentation index 303,304 and the inflection point in the photoplethysmographic digital volume pulse 239 in response to salbutamol inhalation have been used as measures of endothelial function. However, both of these techniques have been found to be much less reproducible in children when compared with brachial arterial flow-mediated dilation. 305 The upper and lower limb pulse wave velocity responses to reactive hyperaemia in the hand and foot have also been used to assess endothelial function. 306
The change in digital pulse volume amplitude during reactive hyperaemia, so-called reactive hyperaemia peripheral arterial tonometry, has also been used to assess peripheral microvascular endothelial function 307,308 ( Fig. 6-11 ). The pattern of the reactive hyperaemia peripheral arterial tonometry has been shown to mirror that of brachial arterial flow-mediated dilation 307 and be attenuated in adults with coronary microvascular endothelial dysfunction. 308 Recently, the central role for nitric oxide in the augmentation of pulse volume amplitude during reactive hyperaemia in humans has been demonstrated. 309

Circulating Biomarkers
While endothelial function is commonly assessed by determining the effects of endothelium-derived nitric oxide on vessel diameter, blood flow, and arterial stiffness, assay of various circulating biomarkers of endothelial and non-endothelial origins may provide additional information on other aspects of endothelial function and magnitude of endothelial activation. These biomarkers include measures of nitric oxide availability, adhesion molecules, inflammatory cytokines, markers of inflammation and oxidative stress, pro- and anti-coagulants, and indices of endothelial cell damage and repair.
The circulating pool of nitric oxide as a measure of its bioavailability can be assessed by measuring plasma nitroso compounds and nitrite. 310–313 While the exact chemical species of this pool are unclear and their values may be confounded by diet, 314 recent studies showed that plasma nitrite reflects regional endothelial nitric oxide synthase activity. 313 Plasma nitrite reserve during reactive hyperaemia 311 and plasma nitroso compounds 310 have been found to be reduced in the presence of endothelial dysfunction. Reduced nitric oxide availability can also be inferred from elevated levels of the naturally occurring antagonist of nitric oxide synthase, dimethylarginine, 315 which has been shown to be associated with cardiovascular disease and mortality in adults. 316 Activation of endothelial cells upregulates the expression of adhesion molecules. The levels of circulation adhesion molecules, including intercellular adhesion molecule 1, vascular cell adhesion molecule 1, E-selectin, and P-selectin can be determined using commercially available assays. Interaction of the dysfunctional endothelium with circulating leucocytes, in which the adhesion molecules play a crucial role, 317,318 initiates inflammation within the vessel wall. Furthermore, oxidative stress, an important determinant of inflammation, is increasingly recognised to play an important role in compromising endothelial function. 319,320 Thus, the levels of inflammatory markers, including high-sensitivity C-reactive protein, interleukin-6, and tumor necrosis factor–α, and markers of oxidative stress, such as oxidised low-density lipoprotein and 8-iso-prostaglandin F2α, can be used to reflect ongoing endothelial activation and vascular inflammation.
Disturbance of the protective role of the endothelium against thrombosis in endothelial dysfunction is reflected by the imbalance between endothelium-derived tissue plasminogen activator and plasminogen activation inhibitor–1 321 and the release into circulation of von Willebrand factor. 322 Measurement of the dynamic release of tissue-plasminogen activator by agonists such as bradykinin, substance P, methacholine, and desmopressin has been used to assess endothelial function, 323–326 although data on its relationship with cardiovascular risk factors and prognostic values is limited.
There is increasing interest in the role of endothelial microparticles and endothelial progenitor cells as markers and risk factors of endothelial dysfunction and cardiovascular disease. Damage to endothelial cells can result in their detachment into the circulation in entirety or as microparticles. Endothelial microparticles have been found to be increased in vasculitis and atherosclerotic diseases. 327–330 The quantity of circulating endothelial cells and microparticles is therefore a potentially useful marker of endothelial function. Interestingly, recent evidence suggests that endothelial microparticles may contribute directly to endothelial dysfunction. 328,331 With regard to the repair of damaged endothelium, apart from proliferation of local mature endothelial cells, the importance of bone-marrow derived endothelial stems cells and endothelial progenitor cells is increasingly recognised. 332–334 Mobilisation of endothelial progenitor cells is in part nitric oxide–dependent. 335 Furthermore, the number of progenitor cells has been shown to correlate negatively with the cardiovascular risk score and positively with brachial arterial flow-mediated dilation in adults. 336 The level of endothelial progenitor cells may hence be a novel marker of endothelial function, vascular dysfunction, and cardiovascular risk in adults. The usefulness of endothelial microparticles and endothelial progenitor cells as markers of endothelial function in the paediatric population awaits further clarification.
SYSTEMIC ARTERIAL DYSFUNCTION IN CHILDHOOD
Age-related Evolution
Progressive increase in aortic, upper limb, and lower limb pulse wave velocities, as measured by transcutaneous Doppler technique, with age in a cohort of subjects aged 3 to 89 years has been reported. 337 Age-dependent increase in brachio-radial arterial pulse wave velocity has similarly been demonstrated in a cohort of children and adolescents aged 6 to 18 years using the photoplethysmographic technique. 338 Using the area under the ascending aortic pressure-time curve to determine the total stiffness of the arterial tree and assuming a two-element Windkessel model, a non-linear increase in total arterial stiffness in children aged 6 months to 20 years has been demonstrated. 339 Although a nadir of arterial stiffness at around 10 years of age has been reported, 340 this has not been replicated in subsequent studies.
Notwithstanding the influence of the distending pressure on arterial stiffness, previous findings did not suggest that the change in pulse wave velocity with age is entirely due to differences in systemic blood pressure. 337,338 Rather, the gradual increase in arterial stiffness with age is probably related to progressive medial degeneration. With cyclical mechanical stress, fragmentation of the elastin fibres and transfer of stress to the much stiffer collagen fibres inevitably result in progressive increase in vascular stiffness. 341 Furthermore, studies of developmental changes in arterial structure during childhood have demonstrated progressive increase in intimal and medial thickness after birth. 342 Hence, the observed age-related increase in stiffness is likely related to progressive structural changes in the arterial wall during childhood.
In otherwise healthy adult subjects, endothelial function has been shown to deteriorate with aging. 343 Furthermore, progressive endothelial dysfunction appears to occur earlier in men than in women. While puberty has been speculated to be a critical period for the vascular endothelium, 344 this requires further clarification.
Cardiovascular Risk Factors
Childhood obesity has become a global epidemic. In obese children, increased stiffness of the abdominal aorta 345,346 and carotid artery 347 has been demonstrated. Endothelial dysfunction is evidenced by elevated serum biomarkers of endothelial activation 348,349 and impaired brachial arterial flow-mediated dilation. 347,350–352 Studies have further shown that in obese children, endothelial dysfunction improves with exercise training. 353–355 Concomitant cardiovascular risk factors linked with arterial dysfunction often occur in obese children and these include dyslipidaemia, diabetes mellitus, and low-grade inflammation. Indeed, endothelial dysfunction in obese prepubertal children has recently been shown to be related to insulin resistance and markers of inflammation. 356 Obese children who have concomitant metabolic syndrome were found to have stiffer common carotid artery than those without. 357 Furthermore, a strong graded inverse association between number of components of metabolic syndrome and brachial arterial distensibility has been demonstrated. 358 The effects of obesity-related peptides on vasculature are increasingly recognised. 359 Elevations in leptin have been shown to be associated with impaired arterial distensibility in healthy children 360 and in children with type 1 diabetes. 361 The effect of leptin on endothelial function in humans is, however, controversial. 362 Plasma adiponectin, on the other hand, has been shown to correlate with vasodilator response of the forearm microcirculation to reactive hyperaemia. 363,364
In children with heterozygous familial hypercholesterolaemia increased stiffness of the common carotid artery 365,366 and modification of the aortic elastic properties 367 have been demonstrated. Impaired brachial arterial flow-mediated dilation has also been shown in these children and in those with familial combined hyperlipoproteinaemia at as young as 6 years of age. 275,368–370 Endothelial dysfunction is most pronounced in those with a positive family history of premature cardiovascular disease. 371 Early statin and antioxidant vitamins C and E therapy may potentially restore endothelial dysfunction in these children towards normal. 369,370,372 However, the relationship between flow-mediated dilation and low-density lipoprotein cholesterol levels is controversial. 365,368,369 On the other hand, in a population-based study, total and low-density lipoprotein cholesterol levels were found to relate inversely to brachial arterial distensibility, 373 suggesting the possibility that cholesterol levels in the general population during childhood may already be of relevance in the pathogenesis of arterial stiffening.
Children with type 1 diabetes have well-documented endothelial dysfunction, 374–377 which has been shown to improve by folic acid. 378 Arterial stiffening in these children is further suggested by an increase in augmentation index, 379 and has recently been found to be associated with nitric oxide synthase 3 polymorphism. 380 Offspring of parents with type 2 diabetes have a high risk of developing diabetes and atherosclerotic complications. Studies have shown increased aortic 381 and carotid-radial 382 pulse wave velocity in normoglycaemic who are offspring of parents with type 2 diabetes. Impaired brachial arterial flow-mediated dilation has similarly been shown in first-degree adult relatives of type 2 diabetic patients. 383–387 Whether such arterial dysfunction has its onset in childhood is unknown.
Structural alterations of the common carotid artery with intima-media thickening have been found in children and adolescents with a parental history of premature myocardial infarction. 388 In adults who are offspring of parents with premature cardiovascular disease, apart from intima-media thickening, impaired endothelial-dependent vasodilation of brachial artery has also been found. 389,390
Habitual physical activity in children aged 5 to 10 years has been shown to correlate positively with flow-mediated dilation, suggesting that its cardiovascular protective effect may be mediated via the endothelium. 391 In another cohort of 10-year-old children, physical activity was found to correlate inversely with arterial stiffness as assessed by measuring the aorto-femoral and aorto-radial pulse wave velocities. 392
Apart from the traditional cardiovascular risk factors, novel risk factors have also been shown to be associated with arterial dysfunction in children. Associations between increased baseline high-sensitivity C-reactive protein concentrations and the risks of developing cardiovascular disease in adults have been reported. 393–395 Furthermore, in vitro studies showed that C-reactive protein induces adhesion molecule expression in human endothelial cells. 396 In healthy children, serum high-sensitivity C-reactive protein concentrations have been found to have an inverse dose-dependent relationship with the magnitude of brachial arterial flow-mediated dilation. 397 Increased serum high-sensitivity C-reactive protein levels are found in obese adolescents. 351,398 Analysis of the 1999–2000 National Health and Nutrition Examination Survey indeed showed a strong independent association between body mass index and C-reactive protein level even in young children aged 3 to 17 years. 399 However, in children and adolescents who do not have much of an atherosclerotic burden, whether high-sensitivity C-reactive protein is a risk factor or a risk marker requires further clarification.
In children with homozygous homocystinuria, impaired brachial arterial flow-mediated dilation has been demonstrated in those as young as 4 years. 400 In the general paediatric population, findings of elevated homocysteine levels in children with a family history of premature cardiovascular disease are conflicting. 401–403 Although hyperhomocysteinaemia is a risk factor for endothelial dysfunction in middle-aged 404 and elderly 405 subjects, there is as yet no data to suggest a link between arterial dysfunction and serum homocysteine levels in children.
Prenatal Growth Restriction
It has almost been two decades since the report of associations between low birth weight and increased risk of cardiovascular disease. 406 These findings, having been replicated in subsequent studies, 407–409 have formed the basis of the fetal origins hypothesis that implicates the origin of cardiovascular disease from adaptations to an adverse environment in utero. These adaptations have been proposed to cause permanent alterations of cardiovascular structure and physiology through the process of programming.
There is evidence that individuals who are born small are at risk of vascular dysfunction in childhood and adulthood. Arterial endothelial dysfunction has been found in term infants, 299 children, 410 and young adults 411 with low birth weight. A recent study showed elevated uric acid in children with low birth weight and a graded inverse relationship between uric acid and flow-mediated dilation. 412 In children born at term, leanness at birth has been reported to correlate with the lowest endothelium-dependent microvascular responses and the highest carotid stiffness indices. 413 In infants with umbilical placental insufficiency before birth, the increase in afterload has been shown to result in a decrease in aortic distensibility during the neonatal period, suggesting an alteration of aortic wall structure. 414 Furthermore, reduced compliance of the aorta and conduit arteries of the legs has been shown to occur in adults born small. 415
The risk of arterial dysfunction for individuals who are born small as a result of prematurity is controversial. An increase in systolic blood pressure has been shown in a cohort of young adults born prematurely, regardless of whether or not they had intra-uterine growth retardation. 416 On the other hand, only individuals who had been preterm babies with intra-uterine growth retardation were found to have impaired brachial arterial flow-mediated dilation. 417 Nonetheless, preterm birth has also been demonstrated to attenuate the association between low birth weight and endothelial dysfunction. 418 With regard to arterial stiffness, reduced aortic wall distensibility and whole-body compliance have been shown in very low birth weight premature infants as early as the neonatal period. 419 Other studies have demonstrated inverse relationships between systemic arterial stiffness and gestational age 420 and birth weight standardised for gestational age. 421
In monozygotic twins with twin-twin transfusion syndrome, the growth-restricted donor twin provides a unique model for studying the effects of differing volume load and increased placental resistance on the developing cardiovascular systems in two genetically identical individuals. A previous study has shown that the peripheral conduit arterial stiffness is increased during infancy in the donor twins. 422 Such vascular programming has been shown to be ameliorated, albeit not completely abolished, by intra-uterine endoscopic laser ablation of placental anastomoses. 423 Even in monozygotic twins without twin-twin transfusion syndrome, the twin with the lower birth weight has been found to have higher systolic blood pressure and pulse pressure and impaired endothelial function in childhood. 424
The mechanism whereby low birth weight is associated with increased arterial stiffness in childhood and adulthood remains unclear. The reported endothelial dysfunction in individuals born preterm and small-for-gestational age 299,410,411,415,417 suggests that functional alteration of arterial tone may contribute to an increase in systemic arterial stiffness. Altered haemodynamics in intra-uterine growth retardation, which result in preferential perfusion of upper part of body, 425 may affect the mechanical properties of the large arteries. Hence, selective carotid arterial atherosclerosis has been found to be more severe in elderly people with the lowest birth weight. 426 Another proposed mechanism is the impairment of synthesis of elastin in the arterial wall. 341 In the donor twin in twin-twin transfusion syndrome, the superimposed circulatory imbalance probably acts synergistically with growth restriction to cause the vascular programming, although the exact mechanism remains to be defined. 422 The exact mechanism of endothelial dysfunction in individuals with low birth weight is even more elusive.
Nutritional Issues
The associations between early growth restriction and arterial dysfunction highlight the potential importance of nutrition in antenatal and early postnatal life on long-term vascular programming. Leeson et al studied in a young adult population-based cohort the relation between duration of breast-feeding and brachial arterial distensibility. 427 They found an inverse relation between duration of breast-feeding and arterial distensibility even after adjusting for current lipid profile, body mass index, and social class. A recent study in 10-year-old children also demonstrated a positive association between breast-feeding and stiffness of the aorto-femoral arterial segment as determined by pulse wave velocity. 392 Despite these findings that evoked much comment, it is important to realise that there is to date little consistent evidence that breast-feeding influences subsequent mortality related to cardiovascular disease 428 and that the current advice on breast-feeding practise has not been altered by these findings.
In children receiving long-term parenteral nutrition, significant increase in elastic modulus of the common carotid artery and impairment of brachial arterial flow-mediated dilation have been demonstrated. 429 While the mechanisms are unclear, infusion of lipid emulsions or high-concentration dextrose has caused endothelial damage and vascular remodeling in animal models. 430,431 It remains unknown whether vascular dysfunction is reversible after reestablishment of enteral feeding.
Childhood Vasculitides
Kawasaki disease, a childhood vasculitis of unknown etiology, is the commonest cause of acquired heart disease in children in developed countries (see Chapter 52 ). The sequelas of inflammation involving coronary and other medium-sized muscular arteries in the acute phase of the disease are well documented. 432–434 Long-term structural alteration and functional disturbance of coronary arteries also have long been known. 432–436 Systemic arterial dysfunction is increasingly recognised in children with a history of Kawasaki long term after the acute illness. Indeed, concerns have been raised regarding the possibility of its predisposition to premature atherosclerosis in adulthood. 437–441
Impaired brachial arterial flow-mediated dilation has been demonstrated in patients, even in those without early coronary arterial involvement, studied at a median of 11 years after the acute illness. 442 Intravenous administration of vitamin C has been shown to improve the impaired flow-mediated dilation. 443 Increased stiffness of the carotid artery 444,445 and brachioradial artery, 441 relating in a dose-dependent manner to the degree of coronary arterial involvement, has also been documented in the long-term follow-up of these patients. By measuring aortic input impedance during cardiac catheterisation, Senzaki et al 446 found that both characteristic impedance and total peripheral arterial compliance were reduced in Kawasaki patients regardless of persistence of coronary artery aneurysms, suggesting an increase in both central and peripheral arterial stiffness. Chronic low-grade inflammation, as reflected by elevated high-sensitivity C-reactive protein, 447,448 in patients with coronary aneurysm formation has been associated positively with carotid arterial stiffness. 447 Indeed, histological evidence of extensive fibro-intimal thickening and infiltration of lymphocytes and plasma cells in the coronary arterial walls has been documented in fatal cases of Kawasaki disease occurring years after apparent resolution of vascular inflammation and in the absence of early detectable coronary arterial abnormalities. 449 In vitro evidence further suggests that chronic activation of the monocyte chemoattractant protein-1/chemokine receptor CCR2 pathway and inducible nitric oxide synthase may play a role in chronic low-grade inflammation in Kawasaki patients. 450 Besides, modulating effects of mannose-binding lectin genotype on arterial stiffness are suggested by findings of patients with intermediate- or low-level expression genotypes having faster brachioradial arterial pulse wave velocity than those with high-level expression genotypes. 451
Limited data exists for systemic arterial dysfunction in other types of childhood vasculitis. Transient impaired forearm vascular endothelium-dependent relaxation has been found in children during the acute phase of Henoch-Schoenlein purpura. 452 In children with polyarteritis nodosa, a chronic vasculitis characterised by recurrent episodes of inflammatory exacerbations, stiffening of the brachioradial artery with amplification during episodes of inflammatory exacerbation has been demonstrated. 338 Endothelial microparticles has also been found to be significantly increased in children with systemic vasculitis and to correlate with disease activity score. 453
Vasculopathies in Syndromal Disorders
Marfan syndrome is caused by mutations of the fibrillin-1 gene. 454 Fibrillin-1, as mentioned earlier, is a matrix glycoprotein and is the principal constituent of microfibrils. Increased aortic stiffness is well documented in patients with Marfan syndrome, as shown by the decreased distensibility and increased stiffness index, 455–463 increased pulse wave velocity, 464 and decreased tissue Doppler-derived systolic and diastolic velocities of the aortic wall. 465 Apart from structural alterations, impaired flow-mediated dilation, possibly related to a defective role of subendothelial fibrillin in endothelial cell mechanotransduction, 466 may also contribute to arterial stiffening. In an animal model of Marfan syndrome, down-regulation of signaling of nitric oxide production in the thoracic aorta has recently been shown to account for endothelial dysfunction. 467 While correlation between fibrillin-1 genotype and aortic stiffness is poor, 468 aortic stiffness has been shown to be an independent predictor of progressive aortic dilation 469,470 and aortic dissection. 470 Beta-blocker therapy 464 and angiotensin-converting enzyme inhibition 471 appear to reduce aortic stiffness, which may in turn slow aortic dilation and delay aortic root replacement. 471
Williams syndrome is a contiguous gene disorder with chromosomal microdeletion at 7q11.23, which encompasses the elastin gene and the genes nearby. 472,473 Haploinsufficiency of the elastin gene has been implicated in the arteriopathy of Williams syndrome. 474 Despite a biological basis for abnormal elastic fibres, results of studies exploring arterial elastic properties in patients with Williams syndrome are controversial. Studies have shown increased stiffness of the ascending aorta and aortic arch and implied that abnormal mechanical property of the arteries may contribute to systemic hypertension, which is common in patients with Williams syndrome. 475,476 By contrast, paradoxical reduction of stiffness of the common carotid artery has also been reported. 477,478 The authors speculated that abnormal elastic fibre assembly in the media may shift the load-bearing structures in the arterial wall to those with a lower elastic modulus.
Systemic arterial abnormalities are common in Turner’s syndrome, which include bicuspid aortic valve, coarctation of the aorta, and aneurysmal dilation of the aortic root. Although histological evidence of cystic medial necrosis has been reported in Turner’s syndrome, 479,480 these findings are not consistently present. Functionally, carotid augmentation index has been found to be increased, explainable in part by the short stature, while carotid-femoral pulse wave velocity and brachial arterial flow-mediated dilation remain normal in these patients. 481,482 It is worthwhile to note, however, that isolated bicuspid aortic valve is associated with progressive dilation of the ascending aorta in both children and adults 483 and increased aortic stiffness. 484,485
Congenital Heart Disease
Aortic medial abnormalities with elastic fibre fragmentation have been identified in intra-operative biopsies and necropsy specimens in a variety of congenital heart disease in patients ranging from neonates to adults. 486 These congenital heart lesions include tetralogy of Fallot with or without pulmonary atresia, truncus arteriosus, complete transposition of the great arteries, coarctation of the aorta, double outlet ventricles, and univentricular hearts. Whether these abnormalities are inherent or acquired is, however, unknown.
Intrinsic histological abnormalities as characterised by medionecrosis, fibrosis, and elastic fragmentation of the aortic root and ascending aorta have been implicated in causing subsequent aortic root dilation in patients after surgical repair of tetralogy of Fallot. 487–489 The potential alterations of aortic mechanical properties have also been studied. In children and adolescents with tetralogy of Fallot, aortic stiffness has been shown to be increased and related to the aortic root dimensions. 490 A subsequent study demonstrated preferential stiffening of the central over peripheral conduit arteries as evidenced by increased heart-femoral pulse wave velocity without concomitant increase in femoral-ankle pulse wave velocity. 491 Importantly, the heart-femoral pulse wave velocity and carotid augmentation index were found to be significant determinants of the size of sinotubular junction, suggesting that central arterial stiffening may contribute to progressive aortic root dilation in these patients.
In transposition of the great arteries, abnormal aorticopulmonary septation has been hypothesised to be associated with events in elastogenesis. 492,493 As described earlier, medial abnormalities have been found in this congenital heart anomaly. 486 In patients undergoing two-stage anatomical correction, decreased distensibility of the neoaorta has been thought to be related to pulmonary arterial banding. 494 Nonetheless, even after one-stage arterial switch operation, impaired distensibility of the neoaorta has similarly been found. 495 Recent studies further documented increased stiffness index of the carotid artery in patients after both atrial and arterial switch operations, 493,496 suggesting that impaired elastogenesis may be an intrinsic component of this congenital anomaly.
Structural abnormalities of the aortic segment proximal to the site of aortic coarctation are characterised by increase in collagen and decrease in smooth muscle content. 497 Functionally, impaired flow-mediated dilation, reduced nitroglycerine-induced vasodilation, and increased pulse wave velocity were found to be confined to conduit arteries proximal to the site of coarctation despite successful surgical repair. 498–500 The distensibility of the aortic arch has likewise been shown to be significantly lower than that of the distal thoracic aorta. 501 Furthermore, analysis of the ascending pressure waveform obtained during cardiac catheterisation revealed enhanced aortic pressure wave reflection as evidenced by a short time to inflection point and increased augmentation index. 502 This has been hypothesised to be related to a major reflection point at the site of coarctation repair. The finding of an inverse relationship between the magnitude of brachial artery vasodilation response to nitroglycerine and 24-hour systolic blood pressure implicates a possible role of reduced vascular reactivity in the development of systemic hypertension and left ventricular hypertrophy in patients late after successful repair of coarctation. 500 The importance of early coarctation repair on possible prevention of late vascular dysfunction is highlighted by the inverse relationships found between age at repair and stiffness and vascular reactivity of the precoarctation arterial segments. 499,501,503 Nonetheless, the results of a recent study suggest that impaired elastic properties of the prestenotic aorta may be a primary abnormality, as evidence by increased ascending aortic stiffness index in neonates with coarctation even pre-operatively. 504
Endothelial dysfunction has also been associated with congenital heart anomalies. In adults with cyanotic congenital heart disease, impaired forearm blood flow response to intra-arterial infusion of acetylcholine has been shown. 505 The cause is probably multi-factorial, being related to reduced nitric oxide production, 506 hypoxaemia, 507 and secondary erythrocytosis. 508 Altered levels of biomarkers of endothelial activation have been shown in a small cohort of children and adolescents with univentricular hearts who have undergone cavopulmonary connection. 509 In patients after the Fontan operation, reduced brachial arterial flow-mediated dilation, 510,511 and, in a subset, impaired nitroglycerine-induced vasodilation 511 have been demonstrated. A negative correlation, albeit weak, was found in post-Fontan patients between flow-mediated dilation and serum levels of nitric oxide pathway inhibitors, asymmetric and symmetric dimethlyarginine. 510 Furthermore, those on angiotensin-converting enzyme inhibitor tended to have better endothelial function. The usefulness of angiotensin-converting enzyme inhibitor in the post-Fontan state nonetheless remains debatable.
Systemic Diseases
Inflammation plays a pivotal role in the pathogenesis of atherosclerosis and cardiovascular disease. 512 The association between low-grade chronic inflammation and endothelial dysfunction has been discussed earlier. Rheumatoid arthritis and systemic lupus erythematosus provide clinical models for determining relationships between chronic systemic inflammation and arterial stiffness and endothelial dysfunction. In adults, rheumatoid arthritis has been associated with increased arterial stiffness 513–518 and endothelial dysfunction. 519–522 In the only available study of children with juvenile rheumatoid arthritis to date, Argyropoulou and colleagues reported increased pulse wave velocity and reduced distensibility of the aorta, 523 as determined by phase contrast magnetic resonance, although these parameters were not found to be related to disease activity parameters. Few studies have evaluated arterial function in children with systemic lupus erythematosus. In women with systemic lupus erythematosus, brachial artery flow-mediated dilation has been found to be impaired 524,525 and carotid arterial stiffness increased. 518,526 In adolescents and young adults with paediatric onset lupus, endothelial function has been found to be normal. 527 However, a recent study demonstrated increased carotid arterial stiffness, which is associated with left ventricular hypertrophy and subclinical left ventricular dysfunction. 528
Apart from intrinsic chronic inflammatory stimuli, acute exposure to extrinsic inflammatory insult in children who had an acute infection or are convalescing from an infection in the previous 2 weeks has been shown to cause transient impairment of brachial arterial flow-mediated dilation. 529 The endothelial dysfunction was found to recover in most but not all of the children studied at follow-up 1 year later. Possible mechanisms include a direct effect of virus 530 or an indirect effect through inflammatory cytokines on endothelial function. Apart from common acute childhood infections, chronic infection with human immunodeficiency virus in children has been associated with arterial dysfunction. The functional alteration is characterised by impaired brachial arterial flow-mediated dilation 531,532 and increased elastic modulus of the carotid arterial wall. 531 Additionally, endothelial dysfunction was found to be more pronounced in children receiving protease inhibitor therapy. 532 Whether these vascular changes are related to metabolic abnormalities such as dyslipidemia and insulin resistance secondary to antiretroviral therapy 533,534 is uncertain. On the other hand, the direct virus-induced and indirect cytokine activation of endothelium 531 may account for the observed arterial dysfunction.
In adults with chronic renal failure, premature atherosclerosis is a major cause of morbidity and mortality. Recent evidence suggests that children with end-stage renal failure are similarly subjected to increased risk of cardiovascular disease. 535 Increased aortic pulse wave velocity and carotid arterial stiffness have been shown to be an independent predictor of cardiovascular mortality in adults with end-stage renal failure. 186,187,536 In children on haemodialysis, increased carotid-femoral pulse wave velocity and augmentation index have also been demonstrated, 537 although the prognostic significance of these indices in children is unknown. Even after successful renal transplantation, the carotid arterial stiffness in children and adolescents remains elevated and is found to be associated with higher daytime systolic blood pressure load and receipt of cadaveric kidney. 538 Apart from arterial stiffening, endothelial dysfunction has been shown in children with chronic renal failure, even in the absence of classic cardiovascular risk factors, 539 and in those after transplantation. 540 Limited evidence suggests that oral folic acid, but not l -arginine, may improve endothelial function in children with chronic renal failure by lowering homocysteine level and increasing resistance of low-density lipoprotein to oxidation. 541,542
Increased iron store has been linked to the risk of atherosclerosis. 543 Iron-overloading in patients with β-thalassaemia major results in alterations of arterial structures with disruption of elastic tissue and calcification. 544,545 Functional disturbance of human vascular endothelial cells when being incubated with thalassemic serum has further been demonstrated in vitro. 546 In adolescent and adult patients with β-thalassaemia major, increased stiffness of the carotid artery, brachioradial artery, and aorta has been shown in vivo. 547,548 Importantly, systemic arterial stiffening is inversely related to brachial arterial flow-mediated dilation and positively with left ventricular mass and carotid intima-media thickness. 547,549 In a mouse model of β-thalassaemia major, the Pourcelot index, a parameter that depends on arterial compliance, downstream vascular resistance, and total peripheral vascular resistance was found to be increased. 550 This result corroborates the findings of elevated pulsatile and static afterload in patients with β-thalassaemia major. 551 Apart from oxidative damage related to iron overload, the cell-free haemoglobin in haemolytic disease has also been implicated in mediating vascular dysfunction by limiting nitric oxide availability. 552
Sleep-related disorders are common in children and may potentially affect arterial function. While relationship between snoring and cardiovascular complications in adults has been controversial, 553–558 in children with primary snoring, higher daytime systemic blood pressure and increased brachioradial arterial stiffness has been reported. 559 In adults with obstructive sleep apnea, increased carotid-femoral pulse wave velocity and brachial-ankle pulse wave velocity have been reported. 560,561 Acute increase in radial arterial stiffness during episodes of apnea has also been shown. 562 While the exact mechanisms are unknown, hypoxia, increased vasoconstrictors, enhanced vasoconstrictor sensitivity, and increased sympathetic tone might account for the alteration of arterial elastic properties. 556–558,560,563,564 Endothelial dysfunction has been shown in adults with obstructive sleep apnea, 565–567 which is potentially reversible by nasal continuous positive airway pressure. 568,569 Whether arterial dysfunction exists in paediatric patients remains to be clarified.
In children with MELAS (mitochondrial myopathy, encephalopathy, lactic acidosis, and stroke), the level of l -arginine has been found to be significantly lower during stroke-like episodes and associated with reduced brachial arterial flow-mediated dilation. 570–572 Prolonged l -arginine supplementation has been shown to reduce the stroke-like episodes and normalise endothelial dysfunction. 571,572
Genetic Considerations
The genetic aspect of arterial stiffness is increasingly being unveiled. The phenomenon of arterial stiffening in genetic syndromes associated with mutations or deletion of genes encoding structural proteins of the arterial wall has been alluded to earlier. Marfan and Williams syndromes are examples whereby genes exert influence on systemic arterial stiffness. Studies have demonstrated significant heritabilities of common carotid artery stiffness, augmentation index, and carotid-femoral pulse wave velocity in different ethnic populations. 573–576 Microarray analysis of the transcriptome of aortic specimens obtained from adults with coronary artery disease has revealed correlations between aortic stiffness and expression of two groups of genes, namely, those associated with cell signaling and those associated with mechanical regulation of vascular structure. 577 Indeed, the influence of matrix metalloproteinase-3 and -9 genotypes on aortic stiffening in healthy elderly subjects has been reported. 578,579 Studies have also related arterial stiffness to polymorphisms of candidate genes, including those involved in the renin-angiotensin-aldosterone system 580–582 and endothelin receptors. 583 In adults with coronary artery disease, fibrillin-1 genotype is associated with aortic input and characteristic impedance and disease severity. 584 Whether fibrillin-1 genotype is similarly associated with aortic stiffening in healthy adults is, however, controversial. 585,586 In children, gene polymorphism of the mannose-binding lectin, a body defence molecule, has been found to exert modulating influence on arterial stiffness after vasculitic damage in patients with a history of Kawasaki disease, as discussed earlier, 451 while arterial stiffening in children with type 1 diabetes is associated with nitric oxide synthase 3 polymorphism. 380 Recently, a genome-wide scan identified linkage of carotid-femoral pulse wave velocity to several separate genetic loci in humans. 576
Potential genetic contribution to endothelial dysfunction is evidenced by studies demonstrating that young individuals with a family history of cardiovascular disease have impaired endothelial function 389,390 and that polymorphisms of angiotensin-converting enzyme 587 and endothelial nitric oxide synthase genes 588 may influence endothelial function. In children, endothelial dysfunction has been documented in single-gene disorders including homocystinuria 400 and familial hypercholesterolemia. 275,368–370 In a large community-based sample, the estimated heritability of brachial artery flow-mediated dilation has been shown to be modest. 589 While polymorphisms of genes encoding factors involved in the regulation of nitric oxide synthesis have been implicated in endothelial dysfunction, 590 further studies are warranted before any conclusions can be drawn.
Clinical Implications
The prognostic implications of arterial dysfunction in adults have been alluded to earlier. In children and adolescents, the predictive value of these measures of arterial dysfunction for future cardiovascular events is unclear. It appears unrealistic, however, to assess prognostic value of indices of arterial function in childhood only in terms of end-points such as cardiovascular morbidity and mortality. Structural surrogate measures of atherosclerosis, such as carotid intima-media thickness, are potentially useful alternatives. 591 Indeed, increased carotid intima-media thickness has been associated with several of the aforementioned paediatric conditions including familial hypercholesterolaemia, 592–594 type 1 diabetes, 374,594 family history of premature myocardial infarction, 388 children infected with human immunodeficiency virus on antiretroviral therapy, 595 obese children with insulin resistance, 596 and thalassaemia major. 549 Nonetheless, whether arterial stiffening and endothelial dysfunction represent genuine childhood cardiovascular risk factors awaits further clarification.
Early identification of arterial dysfunction that potentially precedes and induces atherosclerotic changes provides a window for early intervention. The potential beneficial effects on endothelial function of folic acid in children with renal failure, 541,542 antioxidant vitamins and statins in those with familial hypercholesterolaemia, 369,371,372 vitamin C in those with Kawasaki disease, 443 and exercise training in obese children 353,597 were alluded to earlier. In patients with Marfan syndrome, β-blocker therapy 464 and angiotensin-converting enzyme inhibition 471 appear to reduce aortic stiffness. Longitudinal studies are required to determine whether improvement of arterial function will be translated into clinical benefits. In healthy children, whether such lifestyle and dietary modifications have any effects on the prevention of arterial dysfunction remains to be substantiated.
Given that the systemic arterial system receives and distributes output from the systemic ventricle, satisfactory performance of the systemic ventricular pump depends on not only its intrinsic properties but also its optimal interaction with the systemic circulation. Dysfunction of either of the components of the cardiovascular system would inevitably affect performance of the other. This issue of ventriculo-arterial interaction is discussed in the following section.
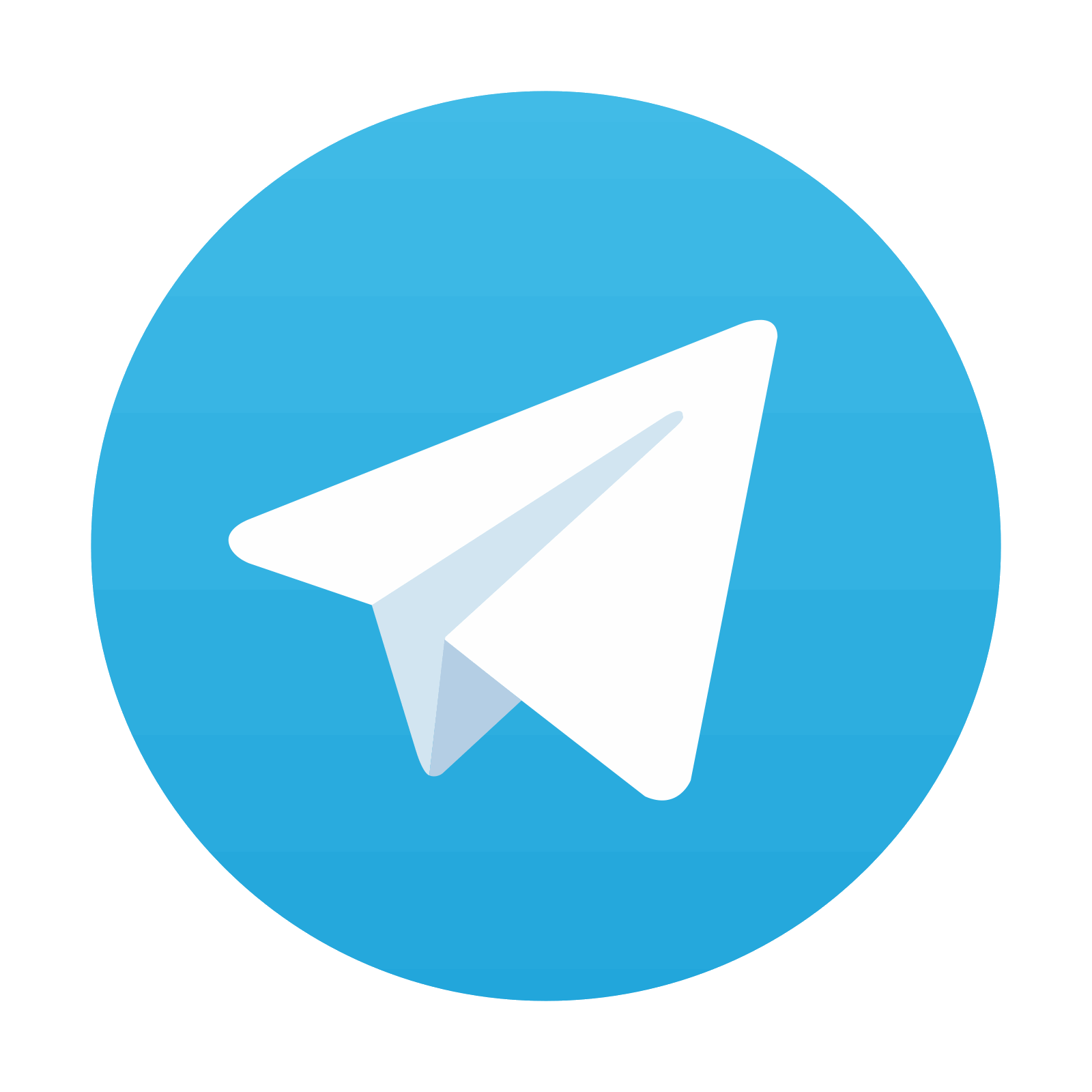
Stay updated, free articles. Join our Telegram channel
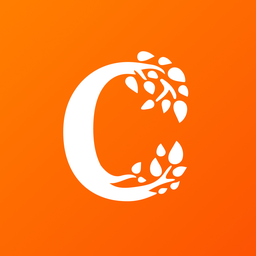
Full access? Get Clinical Tree
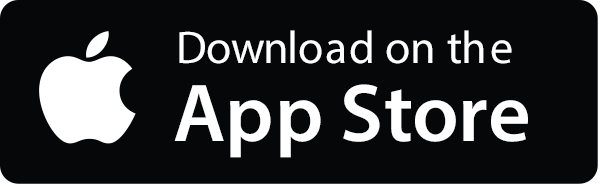
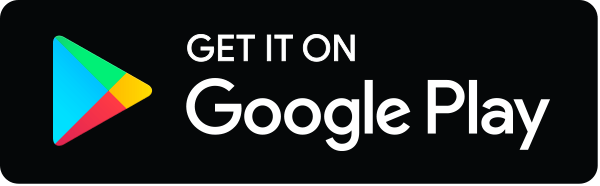