Fig. 5.1
Signaling pathways of α- and β-adrenergic receptors. Note that α2AR is not expressed in cardiomyocytes. β2AR can be coupled with both Gαs and Gαi. Other receptors are coupled with Gαq, including angiotensin II receptor type 1; acetylcholine M1, M3, and M5 muscarinic receptors; vasopressin receptors 1A and 1B; calcitonin receptor; 5-HT2 serotonergic receptors; histamine H1 receptor; and metabotropic glutamate receptor, Group I. Other receptors are coupled with Gαi, including M2 and M4 muscarinic receptors; adenosine A1 and A3 receptors; GABAB receptor; histamine receptors H3 and H4; opioid and nociceptin receptors δ, κ, and μ; apelin receptors; cannabinoid receptors CB1 and CB2; niacin receptors NIACR1 and NIACR2; melatonin receptors MT1, MT2, and MT3; Ca2+-sensing receptor; chemokine CXCR4 receptor; prostaglandin receptors EP1, EP3, FP, and TP; serotonin receptors 5-HT1 and 5-HT5; somatostatin receptors sst1, sst2, sst3, sst4, and sst5; dopamine receptors D2, D3, and D4; and glutamate receptors (mGluR2, mGluR3, mGluR4, mGluR6, mGluR7, and mGluR8). Other receptors are coupled with Gαs, including arginine vasopressin receptor 2; adenosine receptor types A2a and A2b; dopamine receptors D1-like family (D1 and D5); 5-HT receptor types 5-HT4 and 5-HT7; histamine H2 receptor; gastric inhibitory polypeptide receptor; glucagon receptor; corticotropin-releasing hormone receptor; FSH receptor; thyrotropin receptor; parathyroid hormone receptor 1; melanocortin receptor MC1R, MC2R (ACTH receptor), MC3R, MC4R, and MC5R; trace amine-associated receptor 1; prostaglandin receptor types D2 and I2; luteinizing hormone/choriogonadotropin receptor; secretin receptor; box jellyfish opsin; and calcitonin receptor and calcitonin gene-related peptide receptor. AC adenylyl cyclase, AR adrenergic receptor, DAG diacylglycerol, NOS nitric oxide synthase, IP 3 inositol 1,4,5-trisphosphate, PIP 2 phosphatidylinositol 4,5-bisphosphate, PKA protein kinase A, PKC protein kinase C, PLC phospholipase C
There are two classes of ARs: αAR and βAR. Phenylephrine is considered a selective pharmacological agonist of αAR, whereas isoproterenol is a nonselective agonist for βAR [24]. The α1AR subfamily (a Gq-coupled receptor) consists of three highly homologous subtypes, including α1A-, α1B-, and α1D-AR [25]. The α2AR subfamily (coupled to Gi) comprises three subtypes: α2A-, α2B-, and α2C-AR [26]. Some species other than humans express a fourth α2D-AR as well [27]. In the βAR family there are three receptor subtypes: β1AR is found at its highest levels in the heart [28], β2AR is distributed extensively throughout the body [29], and β3AR is mainly expressed in the white and brown adipose tissue [30]. All three βARs couple primarily to Gαs and subsequent cAMP-related pathways, although under certain conditions can also couple to Gαi [31]. β2AR and β3AR signaling can also occur via G protein-independent mechanisms [17, 32]. In particular, cardiac β3AR causes negative inotropic effects mainly mediated by activation of nitric oxide (NO) synthase (Fig. 5.1), serving thereby as a brake in sympathetic overstimulation. These paradigms of signaling can be observed in the same cell type based on the functional state of the cell. Henceforth, the response to GPCR stimulus can be modified by various conditions, including chronic stimulation, acidosis, cell hypoxia, and aging [33].
5.4 Functional Involvement of Adrenergic Receptors in the Aging Process
The age-associated decrease in catecholamine responsiveness in the elderly is well established and widely acknowledged. In particular, an age-related reduction in βAR sensitivity and density has been clearly shown in the myocardium and has been essentially attributed to downregulation and impaired coupling of βARs to adenylyl cyclase [34]. More specifically, the age-linked decline in cardiac βAR response appears to be principally due to a downregulation of β1ARs, as reported in aged explanted human hearts [35]. Additionally, a decreased sensitivity of βARs, measured by isoproterenol-induced changes in the catecholamine-stimulated adenylyl cyclase activity, has been demonstrated in the myocardium and in pulse rate and blood pressure [29]. βAR compartmentalization may also participate in the age-associated decreased βAR responsiveness [36, 37]. Indeed, whereas β1ARs are widely distributed on the plasma membrane, β2ARs are usually located in the transverse tubules, invaginations of the plasma membrane containing several proteins that couple membrane depolarization (excitation) to calcium-mediated myofilament shortening (contraction) [29, 38]. Henceforward, the peculiar localization of β2AR in cardiac cells leads to the generation of spatially restricted cAMP production, affecting Ca2+-dependent proteins that control the contraction of myofilaments [39]. Interestingly, a disrupted localization of β2AR has been recently described in chronically failing cardiomyocytes, with considerable functional consequences [40]. Numerous conditions presenting a depressed cardiac function elicit activity from the sympathetic nervous system that ultimately increases cardiac output and diverts blood flow to critical organs. The main actors of this system are catecholamines, whose release is strictly orchestrated by the GPCR system, relating the adrenal gland and the heart [41]. Most of the modifications in the sympathetic nervous system occurring with aging, including decreased βAR responsiveness, increased circulating catecholamines, and overall hyposensitivity to adrenergic stress, are also common in patients with heart failure. Moreover, young people are more reactive to isoproterenol-induced increase in blood flow than elderly subjects [42].
Vascular tone is finely regulated by the intimal (endothelial cells, EC) and the medial (vascular smooth muscle cells, VSMC) layers [14, 43], and both EC and VSMC express β2AR [44, 45]. Therefore, such a receptor is expected to play a key role in the reduced vasoreactivity observed in the elderly [46]. Of interest, the age-related decline in β2AR function and successive cAMP generation is a common factor to several cardiovascular disorders, including hypertension, atherosclerosis, vascular insufficiency, and orthostatic hypotension, all conditions with significant mortality and morbidity [15, 47–49]. The increased incidence of atherosclerosis and restenosis in aged people may also rely on the age-associated deterioration in βAR-mediated cAMP production, since cAMP may inhibit VSMC proliferation [50]. A reduced responsiveness with age has been also reported for αAR in healthy subjects [51], with potential implications for reduced muscle blood flow and augmented blood pressure during exercise [52].
5.5 Adrenergic Signaling in Cardiovascular Aging and Heart Failure
The sympathetic nervous system exerts various effects on the cardiac physiology, including increase in atrioventricular conduction (positive dromotropy), heart rate (positive chronotropy), cardiac contractility (positive inotropy), and cardiac relaxation (positive lusitropy). Likewise, it plays a crucial role in the regulation of vascular tone due to its ability to control at the same time both peripheral resistances and cardiac output [53].
Heart failure is a chronic clinical syndrome in which the heart is incapable of pumping a sufficient supply of blood to meet the metabolic requirements of the body or requires elevated ventricular filling pressures to accomplish this goal. Heart failure leads to a debilitating illness characterized by poor exercise tolerance and chronic fatigue, representing one of the most important causes of morbidity and mortality worldwide. Notwithstanding considerable advances in its treatment, heart failure still represents a severe social and clinical burden [54, 55]. A complex neurohormonal regulatory system exists between the heart and multiple organ systems, including feedback loops mediated through a variety of vasoactive substances secreted by the adrenals, kidneys, lungs, and endothelium. Perturbations of function in any of these organs affect the others. Accordingly, the cardiovascular system must be perceived in a dynamic manner, continually adapting in order to optimize organ perfusion. During heart failure, diverse neurohormonal mechanisms get triggered in order to maintain cardiac output. Heart failure is indeed a progressive disease that begins long before symptoms or signs become evident. It is initially characterized by a complex adaptive neurohormonal activation, which includes the nervous system, renin–angiotensin–aldosterone system, natriuretic peptides, endothelin, and vasopressin. These and other regulatory mechanisms are required to compensate for cardiac dysfunction [56]; however, when the left ventricular dysfunction persists, the process progressively becomes maladaptive, eventually leading to increased mechanical stress on the failing heart, causing detrimental electrical and structural events, further impairment of systolic and diastolic function, and progressive cardiac fibrosis and apoptosis [57] (Chap. 3). Thus, β-blockers, angiotensin-converting enzyme inhibitors, angiotensin II AT1 receptor blockers, and mineralocorticoid receptor antagonists represent cornerstones for the treatment of patients with failing hearts [58] (Chaps. 8, 36, and 38).
The central part of the adrenal gland, called adrenal medulla, constituted of groups of adrenergic and noradrenergic chromaffin cells and in the minor part of ganglionic neurons, is the main source of catecholamines. Here the chromaffin cells secrete around 80 % adrenaline (epinephrine) and 20 % noradrenaline (norepinephrine), whereas this proportion is reversed in the sympathetic nerves, which contain predominantly noradrenaline [41]. The adrenergic and noradrenergic secretion in different groups of chromaffin cells relies on the different α2AR subtypes’ expression [59]. The adrenal gland can be compared to a specialized sympathetic ganglion, receiving inputs from the sympathetic nervous system via preganglionic fibers. However, it directly secretes neurohormones into the bloodstream. Indeed, chromaffin cells are postganglionic sympathetic neurons that have lost part of their characteristics as axons and dendrites and are able to secrete their hormones into the blood by exocytosis. The suggestive link between the adrenal gland and the heart has become quite interesting and stimulating in the last few years, with several studies investigating the molecular mechanisms underlying such a complex relationship, especially in the pathophysiology of heart failure [41].
Adrenaline and noradrenaline generally have similar effects, although they differ from each other in certain actions. In particular, noradrenaline constricts almost all blood vessels, while adrenaline causes constriction in many networks of minute blood vessels but dilates the vessels in the skeletal muscles and the liver [60]. Both sympathomimetic agents increase heart rate and myocardial contractility, thereby augmenting cardiac output and blood pressure [61]. Sympathetic overdrive observed in heart failure correlates with a higher risk of arrhythmias and left ventricular dysfunction [62]. Plasma concentrations of noradrenaline are negatively associated with survival in heart failure patients [63]. Augmented levels of circulating catecholamines can cause myocardial damage via an enhancement of the cardiac oxygen request and by increasing peroxidative (and lipoperoxidative) metabolism and ensuing free radical production [64, 65]. They lead to structural alterations in the myocardium, including focal necrosis and inflammation, increased collagen deposition, and subsequent interstitial fibrosis [66, 67]. Noradrenaline can also increase cardiac oxygen consumption and cause apoptosis, ultimately leading to dilated cardiomyopathy [68, 69].
At the vascular level, systemically circulating or locally released catecholamines [44] trigger two main classes of ARs: α1AR and β2AR, causing vasoconstriction and vasodilatation, respectively [45, 70]. With aging, such a fine equilibrium is progressively shifted toward increased vasoconstriction, most likely due to a defective vasodilatation in response to βAR stimulation. Supporting this hypothesis, βAR agonist administration in the human brachial artery induces vasodilatation, and this response appears to be attenuated in hypertensive patients [42]. The mechanistic role of β2AR in the vasculature is also corroborated by the fact that genetic variants of β2AR causing excessive desensitization have been shown to lead to reduced vasodilatation, promoting the development of atherosclerosis [71].
Increased basal levels of circulating catecholamines have been observed both in heart failure and with advancing age [72], mirrored by a decrease in the number of high-affinity βARs [73], suggesting that these alterations might be due to βAR desensitization rather than actual reduction in βAR density [74]. As mentioned above, βAR affinity for the ligand is mainly dependent upon GPCR phosphorylation, which in turn is in the domain of GRKs [75]. Modulation of sympathetic nervous signaling via GRK-mediated downregulation of βARs in the heart plays a key role in heart failure. In particular, heterozygous GRK2 knockout mice display augmented cardiac contractility and function, whereas transgenic mice overexpressing cardiac GRK2 exhibit decreased myocardial function due to βAR dysfunction [76].
GRK2 expression and activity increase in vascular tissue with aging [20]. Equally important, an impairment in βAR-mediated vasorelaxation has been observed in hypertensive patients [42] and in animal models of hypertension [16, 77]: such alteration has been related to the increased GRK2 abundance and activity. Transgenic overexpression of GRK2 in the vasculature causes impaired βAR signaling and vasodilatative response, eliciting a hypertensive phenotype in rodents. This aspect has been confirmed in humans: GRK2 expression correlates with blood pressure and impaired βAR-mediated adenylyl cyclase activity [20]. Besides, genetic variants of the β2AR affecting its translational efficiency have been associated with longevity [78]. The decrease in βAR-mediated responses has been attributed to different mechanisms, including an attenuation of protein kinase A (PKA) activation, an impaired generation of cyclic AMP, a decreased receptor density, and a less efficient coupling to adenylyl cyclase [29]. Variations in cyclooxygenase expression and vasoactive prostanoid levels have also been suggested as potential mechanisms. However, currently there is not a single molecular or cellular factor that can fully explain the decline in βAR function. Nevertheless, the etiology seems to be most likely associated with alterations in the ability of βAR to respond to agonists at the cellular level.
5.6 Therapeutic Implications: Pharmacologic and Non-pharmacologic Options
5.6.1 β-Adrenergic Receptor Blockade
Based on receptor-level activity, β-blockers can be classified into three generations (Table 5.1): (1) first generation, nonselective drugs that block both β1AR and β2AR; (2) second generation, cardioselective agents, with higher affinity for β1AR; and (3) third generation, β-blockers with vasodilatative properties, mediated by α1AR blockade, β2AR agonism, or NO synthesis. Both selective and nonselective β-blockers have negative inotropic and chronotropic effects. The reduced inhibitory effect on β2ARs makes the selective β-blockers less likely to cause peripheral vasoconstriction. Therefore, exercise performance may be impaired to a lesser extent by β1AR selective drugs, at least in part because β2AR blockade tends to blunt the exercise-induced increase in skeletal muscle blood flow [3]. Exercise training has been shown to improve βAR signaling and function, augmenting cardiac inotropic reserves, increasing peak oxygen uptake, and restoring normal sympathetic outflow and circulating catecholamine levels [3, 98].
Table 5.1
Classification and pharmacology of β-adrenergic receptor blockers
β1AR selectivity | α1AR blockade | ISA | MSA | Half-life (hours) | Bioavailability (%) | Comment | Reference | |
---|---|---|---|---|---|---|---|---|
First generation (nonselective) | ||||||||
Carteolol | – | – | + | – | 6–8 | 85 | Used to treat glaucoma | [79] |
Levobunolol | – | – | – | – | 20 | 75 | Used to treat glaucoma | [80] |
Nadolol | – | – | – | – | 10–20 | 35 | Low lipid solubility | [81] |
Penbutolol | – | – | + | – | 5–6 | 90 | 5-HT1A antagonist | [82] |
Pindolol | – | – | ++ | ± | 3–4 | 75 | Long-lasting hemodynamic effects | [83] |
Propranolol | – | – | – | ++ | 3–5 | 25 | Treatment of performance anxiety | [84] |
Sotalol | – | – | – | – | 12 | 95 | Inhibition of K+ channels | [85] |
Timolol | – | – | – | – | 3–5 | 65 | Reduces intraocular pressure | [86] |
Second generation (cardioselective) | ||||||||
Acebutolol | + | – | + | + | 3–4 | 40 | Used in Smith–Magenis syndrome | [87] |
Atenolol | + | – | – | – | 5–8 | 50 | Almost exclusive renal excretion | [88] |
Betaxolol | + | – | – | ± | 14–22 | 89 | Used to treat glaucoma | [89] |
Bisoprolol | + | – | – | 9–12 | 90 | Minor effects on insulin sensitivity | [90] | |
Esmolol | + | – | – | – | 0.15 | 99 | Ultrashort acting | [91] |
Metoprolol | + | – | – | ± | 3–7 | 12 | Available as succinate or tartrate | [92] |
Third generation (vasodilators) | ||||||||
Bucindolol | – | + | ± | – | 3–4 | 30 | Debatable results in clinical trials | [93] |
Carvedilol | – | + | – | – | 7–10 | 30 | Ameliorates insulin sensitivity | [94] |
Celiprolol | + | – | + | + | 5 | 50 | β2AR agonist | [95] |
Labetalol | – | + | + | ± | 4–6 | 20 | Used to treat high blood pressure | [96] |
Nebivolol | + | + | – | – | 10–30a | 12–96a | Nitric oxide vasodilatatory effect | [97] |
β-Blockers differ in their physicochemical properties. For instance, lipophilic compounds, including metoprolol, carvedilol, nebivolol, and bucindolol, are rapidly adsorbed in the gastrointestinal tract and are extensively metabolized in the liver (first-pass metabolism), resulting in a shorter half-life when compared to other β-blockers [99]. Furthermore, the high lipophilicity leads to an enhanced penetration across the blood–brain barrier, which may justify the increased number of central nervous system-related effects as well as the membrane-stabilizing properties of antiarrhythmic molecules, which are independent of the βAR blockade [100].
The most common adverse events of β-blockers are attributable to the blockade of sympathetic stimulation, resulting in acute or chronic consequences at cardiovascular, metabolic, and respiratory levels [101, 102]. In the heart, acute blockade of catecholamine effects worsens myocardial contractility and induces bradycardia. Starting with low doses and slowly titrating up is a commonly used approach to reduce these risks. Patients may experience worsening of their symptoms during β-blocker titration, often requiring increased diuretic doses. Since a prolonged β-blocker treatment may enhance the sensitivity to catecholamines, an abrupt withdrawal should be avoided. At the respiratory level, β-blockers can cause bronchoconstriction, due to β2AR blockade. Therefore, β-blockers, especially nonselective agents, are contraindicated in patients suffering from asthma or chronic obstructive pulmonary disease. A risk/benefit assessment should be performed in each single patient in order to avoid undertreatment of heart failure [103, 104].
Counteracting adrenergic overdrive through means of βAR antagonists has been shown to reduce cardiac workload and increase O2 sparing in patients with failing heart. However, β-blockers have also noteworthy metabolic implications, including alterations in the lipoprotein profile, namely, a reduction in high-density lipoprotein (HDL) cholesterol and an increase in triglycerides, and a deranged glucose homeostasis, which is essentially attributed to the blockade of β2AR-dependent insulin release from the pancreatic islets of Langerhans [17]. β1AR selective antagonists should thereby be preferred in patients with diabetes and heart failure [49]. The variability in the response to β-blockers has been at least in part attributed to polymorphisms in CYP2D6 gene, which is highly polymorphic in humans [105]. Indeed, many β-blockers are partially or totally metabolized by CYP2D6, and opportune dose modifications should be accurately considered in patients treated with drugs processed by the same CYP isoforms, including antipsychotics and antidepressants [106]. Equally important, half-life and peak plasma concentration are influenced by the formulation of the molecule. For instance, metoprolol is available in two different formulations: metoprolol succinate [107], with a long-lasting action (Metoprolol CR/XL Randomized Intervention Trial in Congestive Heart Failure – MERIT-HF), and metoprolol tartrate [108], which has a short half-life and demonstrated a reduced efficacy when compared to carvedilol (Carvedilol or Metoprolol European Trial – COMET study). The FDA has approved metoprolol succinate for the treatment of subjects with failing hearts [109, 110].
5.6.2 α-Adrenergic Receptor Blockade
As potent vasodilators, α1AR blockers were initially thought to be promising to treat heart failure. However, chronic administration of the α1AR blocker prazosin caused an increase in catecholamine levels. Two clinical trials demonstrated that the hypothesis of using α1AR blockers to treat heart failure was not pursuable. First, in the Veterans Administration Cooperative Study (V-HeFT), patients receiving prazosin experienced worse outcomes than those receiving the combined vasodilator therapy of isosorbide dinitrate and hydralazine [111]. Then, in the ALLHAT (Antihypertensive and Lipid-Lowering Treatment to Prevent Heart Attack Trial) study, the doxazosin arm was terminated early because of the higher incidence of heart failure [112]. Mounting evidence indicates that the central nervous system plays a crucial role in the sympathetic excitation observed in heart failure. Moreover, the association between the degree of sympathetic activation and mortality raised the possibility that a more complete adrenergic blockade might produce better outcome. Since the excitation of central α2AR inhibits the activation of the sympathetic nervous system, such a receptor has been considered as a possible target in the treatment of heart failure [113].
Clonidine is a centrally acting drug with α2AR agonist action that at modest doses has been shown to markedly attenuate cardiac and renal sympathetic tone in patients with failing hearts in a small and short-term clinical study. However, a clinical trial (Moxonidine in Congestive Heart Failure – MOXCON) investigating the centrally acting sympatholytic agent moxonidine had to be terminated early [114] because the drug was associated with an increased mortality (+38 %) and hospitalizations for heart failure and myocardial infarction, despite a significant dose-related reduction in plasma noradrenaline (−18.8 %). These results suggest that peripheral receptor inhibition may be better tolerated than central suppression of the sympathetic nervous system (Chap. 39). A marked sympatholytic effect has been also associated with adverse outcomes in the Beta Blocker Evaluation of Survival Trial (BEST), where patients in which bucindolol induced a significant decrease in noradrenaline levels exhibited a 169 % increase in mortality [115]. Intriguingly, one of the oldest drugs used to treat heart failure, digoxin, which mainly acts by indirectly increasing intracellular Ca2+ available in the sarcoplasmic reticulum, has been shown to modulate the adrenergic nervous system by improving baroreceptor function and decreasing sympathetic tone [116].
5.6.3 RAAS Interruption
The renin–angiotensin–aldosterone axis (RAAS) is another key system involved in the pathophysiology of heart failure, and the degree of its activation correlates with prognosis [117]. Angiotensin II and aldosterone production have been shown to enhance the release (and inhibit the uptake) of noradrenaline at the nerve endings. Plasma aldosterone levels may be elevated as high as 20-fold in heart failure patients, primarily because of augmented production by the adrenal glands following stimulation by the high circulating levels of angiotensin II [118]. Together with its metabolic and electrolyte effects, aldosterone causes endothelial dysfunction, increases plasminogen activator inhibitor-1 levels, and induces the development of cardiac fibrosis, accelerating the remodeling process and disease progression [119, 120]. Undoubtedly, angiotensin-converting enzyme inhibitors and angiotensin II receptor blockers represent cornerstones in heart failure therapy [121] (Chap. 36). Furthermore, two clinical trials (Eplerenone Post-Acute Myocardial Infarction Heart Failure Efficacy and Survival Study (EPHESUS) and Randomized Aldactone Evaluation Study (RALES)) have demonstrated the benefits of aldosterone antagonists in heart failure, probably also related to their effect on the sympathetic nervous system [122, 123].
5.6.4 Non-pharmacologic Options
Recent evidence suggests that the modulation of the sympathetic nervous system can also be achieved via non-pharmacologic routes, including sympathetic denervation, baroreflex sensitization, and vagal nerve stimulation [124, 125] (Chap. 6). However, randomized clinical trials are required to address the potential benefits of these treatments in patients with heart failure. Of note, cardiac resynchronization therapy has been shown to improve sympathetic function in patients with reduced systolic function [57, 126]. Biventricular pacing can indeed reduce cardiac sympathetic nerve activity and revert autonomic remodeling, at least in part by upregulating presynaptic receptor function [127–129].
5.7 Adrenergic System in the Heart: Beyond the Regulation of Contractility
The activation of the sympathetic system leads to noteworthy metabolic responses, including increased gluconeogenesis and lipolysis with subsequent elevated plasma levels of free fatty acids and glucose [47, 130]. Essentially, the extra amount of glucose and free fatty acids can be used by the organism as fuel in times of stress or danger, when increased exertion or alertness is required [131]. Different therapeutic approaches targeting myocardial metabolism have been suggested to regulate metabolic pathways in the failing heart and improve cardiac function and metabolic elasticity [132] (Chap. 17).
During the flight-or-fight response, sympathetic activation causes α1AR-mediated vasoconstriction in less vital vascular beds, such as splanchnic and skin, to divert the blood to the skeletal muscle in exercise. AR activation also mobilizes blood from the capacitance veins, involving α1 and α2 ARs [133]. These acute physiological responses, typical of the stress conditions, are disadvantageous when they become chronic. Actually, a common feature of many pathological conditions involving sympathetic system hyperactivity is the development of metabolic alterations, including insulin resistance, impaired glucose and lipid metabolism, and mitochondrial dysfunction [3, 134]. The myocardium has high metabolic demands, among the highest in the body: with minimal ATP reserves and complete ATP turnover approximately every 10 s, the heart heavily depends on a continuous energy supply [135]. Though, the heart possesses a strategic metabolic flexibility that allows maintaining its function during stressful conditions. The cardiac muscle generates ATP almost exclusively via oxidative phosphorylation by using different metabolic substrates: in healthy state cardiac ATP production mainly relies on free fatty acid oxidation, whereas the relative contribution of glucose increases during stress or injury [136].
< div class='tao-gold-member'>
Only gold members can continue reading. Log In or Register a > to continue
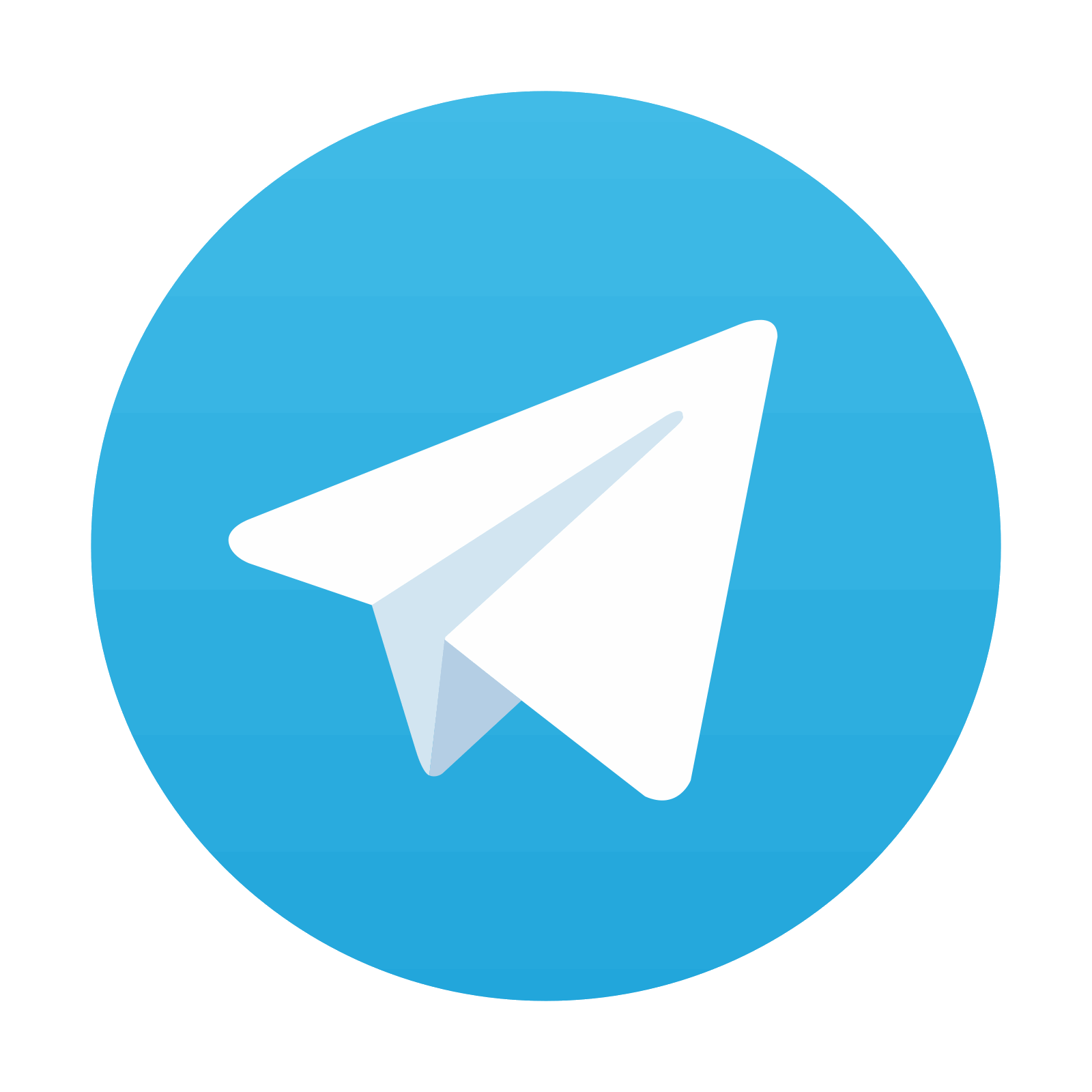
Stay updated, free articles. Join our Telegram channel
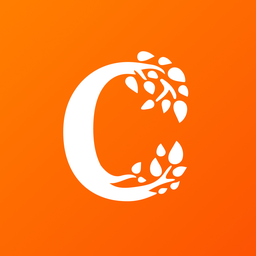
Full access? Get Clinical Tree
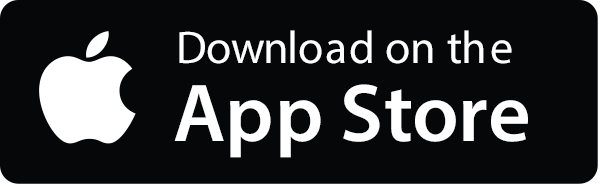
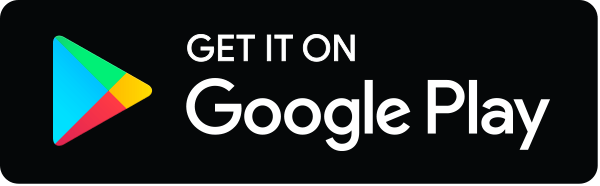