LEARNING OBJECTIVES
Learning Objectives
The student will be able to define static lung compliance as determined using excised lungs inflated by negative pressure.
The student will be able to distinguish between tissue elastic recoil forces and surface tension recoil forces, and interpret hysteresis curves obtained during lung inflations with air, saline, or with air after saline lavage.
The student will be able to summarize the origin, cellular location, and primary constituents of pulmonary surfactant.
The student will be able to describe the major laboratory methods for analyzing amniotic fluid for pulmonary surfactant, including cutoff values for the foam stability index, the fluorescent polarization assay, and the lecithin/sphingomyelin ratio.
Lungs normally resist their own expansion throughout inspiration by means of inwardly directed recoil forces that would collapse them if left unopposed by the chest wall and diaphragm that are recoiling outward. Thus, lung volume at end-expiration and with the glottis open to atmospheric pressure represents the balance point of these directionally opposite forces, and was defined previously as functional residual capacity (FRC). The following discussion will summarize the features of healthy lung tissue that provide its inherent elastic recoil, and the role that pulmonary surfactant plays in modulating that elasticity so that breathing can be both effective and energetically feasible.
ELASTIC PROPERTIES OF LUNG TISSUE
The description in Chap. 4 of gas movement during ventilation introduced the concept of static elastic and surface tension recoil forces. These forces are visually evident on excised healthy lungs that quickly collapse to their minimal volume if the chest is opened to outside air, creating a pneumothorax. Such lungs normally retain air only in large airways and a few clusters of alveoli, having undergone atelectasis. The collapsed lungs are suspended by the trachea within a vacuum jar that can be evacuated to simulate negative intrapleural pressure (PIP) in vivo (Fig. 5.1). As jar pressure is made more negative in small stable increments, the lungs ‘inspire’ air and equilibrate at a new volume until jar pressure is altered again. In this stepwise way, stable changes in total lung volume per unit change in pressure are recorded when dynamic airway resistance is absent, and used to calculate their static compliance (ΔV/ΔP).
When negative pressure is first applied to the collapsed lungs, their volume does not change, that is, their compliance is zero. Then, as the lungs achieve a critical opening pressure (PCO) their compliance rapidly increases and the lungs fill easily. Thus, PCO is the minimal pressure required to open or recruit alveoli and small airways that were previously atelectatic. As pressure inside the jar becomes even more negative (−20 to −30 cm H2O), lung volume approaches a maximum, equivalent to total lung capacity TLC in vivo. Then, as the vacuum is slowly released stepwise toward 0 cm H2O, normal lungs deflate toward residual volume RV. A complete cycle of filling and emptying the lungs shows that their static compliance (ΔV/ΔP) is maximal when lung volume = 50%-70% of total lung capacity during inflation versus 20%-30% of total lung capacity during deflation. Furthermore, a more negative pressure is needed to inflate lungs to a volume than to maintain that volume during the next deflation. This discrepancy between inflation and deflation is termed hysteresis, with an enclosed area that is proportional to the non-recoverable work required during inflation to overcome the recoil properties of air-filled lungs.
What component of static lung recoil causes hysteresis? The answer can be found by repeating the stepwise inflation and deflation of excised lungs with normal saline, NS (0.9% NaCl) instead of air to fill the airways (Fig. 5.2). Doing so yields a similar but not identical pair of curves to those in Fig. 5.1. The first and most obvious difference in compliance ΔV/ΔP between saline-filled lungs and air-filled organ is that less negative pressure is required to achieve both PCO and then TLC, in this example being only −8 cm H2O for total lung capacity with saline versus −20 cm H2O for air. Indeed, a PCO cannot really be demonstrated for saline-filled lungs. Second, regardless of where static compliance is measured during inflation and deflation, it is always greater for saline-filled lungs than for air-filled lungs, that is, the saline ΔV/ΔP curves are steeper. A third difference is the negligible hysteresis in saline-filled lungs. Energetically this means that virtually all ventilatory effort expended to inflate them is recovered during deflation, since saline-filled lungs behave more like an ideal elastic model.
FIGURE 5.2
Static V/P curves for air-filled and saline-filled lungs of a rabbit, and the same lungs lavaged with saline to remove surfactant before reinflation with air. Open circles are inflation. Saline-filled lungs are more compliant (ie, ΔV/ΔP is greater) than air-filled and show no critical opening pressure or hysteresis; both saline-filled and air-filled lungs are more compliant with less hysteresis than lungs that are lavaged and then reinflated.
When these saline-lavaged lungs are reinflated to mimic the original air-filled ΔV/ΔP curves (Fig. 5.2), they are now much less compliant than before lavage, or ‘stiffer’ in the jargon of pulmonary medicine. As a result, a more negative PIP must be applied to achieve both PCO and total lung capacity, which will require more ventilatory effort. Equally significant, lavaged lungs show more hysteresis reflecting the non-recoverable work of breathing. Ventilating such lungs increases the risks of pleural rupture and respiratory muscle fatigue due to the high inflating pressures needed to achieve total lung capacity.
CLINICAL CORRELATION 5.1
The terms compliance, distension, and distensibility may seem synonymous, but clinically their meanings can be quite different. One easy analogy may help. A new rubber balloon is distensible (expandable with gas or liquid) and it also is compliant (its rubber walls resist inflation and assist deflation). A plastic bag from the grocery store is distensible, but it is not compliant. A rubber balloon that is filled and then forgotten will remain distensible but become less compliant as its walls lose their elasticity. Certain diseases affect lungs in such fashion, notably emphysema in which lung elasticity is reduced by proteases released into the alveolar parenchyma by resident macrophages and recruited neutrophils (Chap. 20). Lungs of patients with severe emphysema can be inflated but may retain gas even during a pneumothorax.
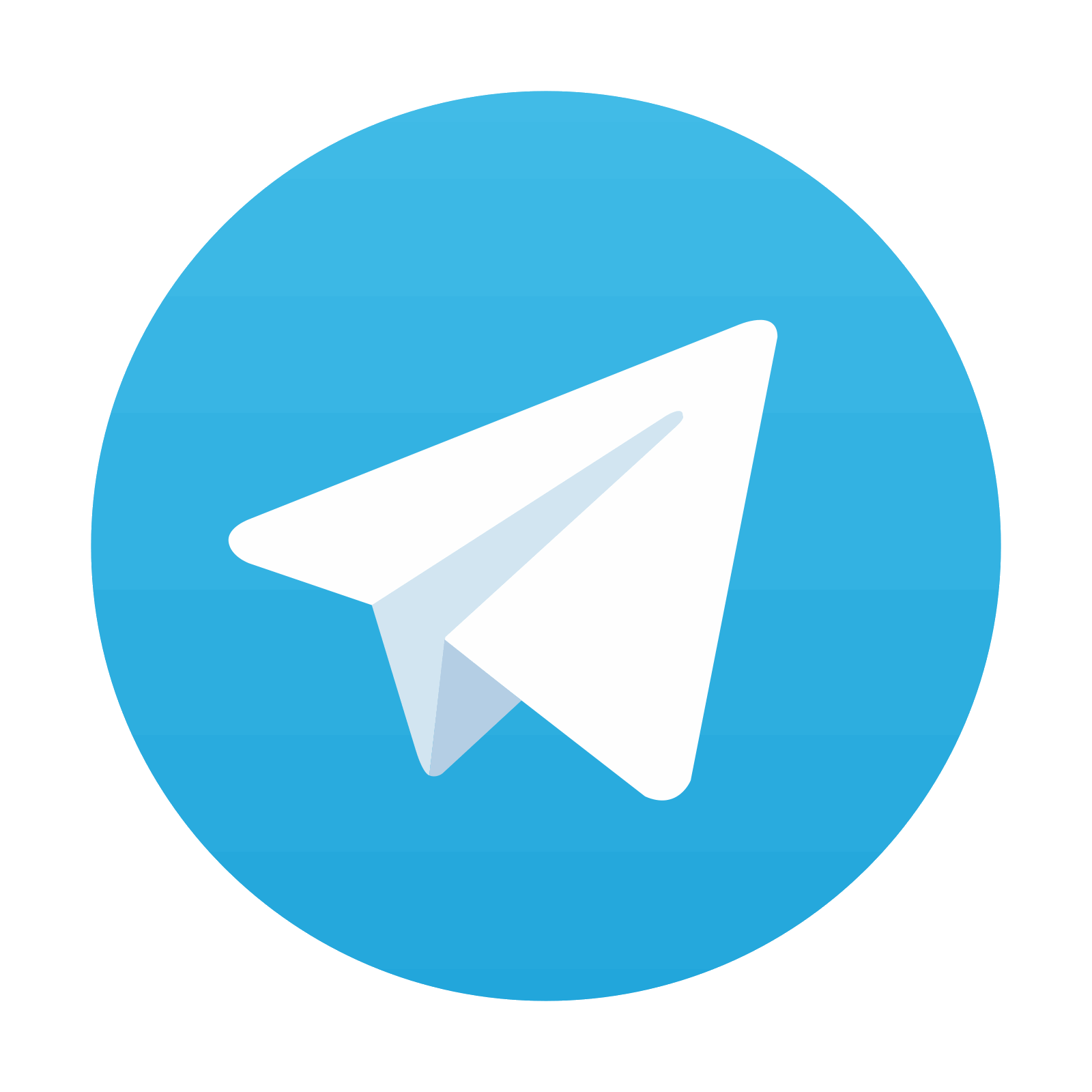
Stay updated, free articles. Join our Telegram channel
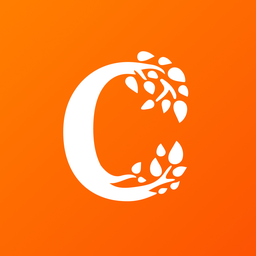
Full access? Get Clinical Tree
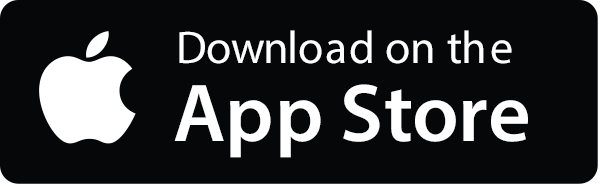
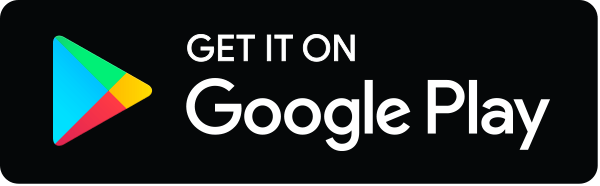