Fig. 17.1
Conditions leading to remodeling of the heart and resulting in atrophy or hypertrophy. Depending on the circumstances, remodeling can be normal or pathologic. Pathologic remodeling is associated with a propensity toward decompensation, ventricular dilatation, systolic dysfunction, and electrophysiologic changes leading to malignant ventricular arrhythmia. Source: Hill JA, Olson EN. Cardiac plasticity. N Engl J Med. 2008;358:1370–80
In the 1960s, a different view of LV hypertrophy and enlargement began to emerge. In accordance with Laplace’s law, which dictates that afterload-induced increases in systolic wall stress are offset by increases in wall thickness, hypertrophic growth of the heart was seen as “compensatory” and, hence, beneficial [9, 10]. Animal models of pressure overload led Meerson to suggest that cardiac growth induced by biomechanical stress plays a protective role, at least in the short term [11]. Moreover, in the 1970s and 1980s, hemodynamic measurements in patients with valvular heart disease provided support for the concept of adaptive hypertrophic growth which, when “inadequate,” could lead to systolic dysfunction [12–14].
Recent clinical studies have called into question the idea that structural changes of the ventricle are adaptive and protective. Progressive LV hypertrophy, enlargement, and cavity distortion over time have consistently been shown to be directly related to the deterioration of LV performance and an increase in mortality and morbidity [15–19], irrespective of the etiology of HF [20].
Current concepts of ventricular remodeling are largely derived from studies on patients and animal models of MI and hypertension [21–24]. Studies by Chanutin and Barsdale [25] on an experimental model of arterial hypertension demonstrated that LV weight and myocyte fiber diameter increased in relation to the severity of hypertension. Janice Pfeffer and her colleagues [26, 27] studied the relationship between LV mass and function over time, in the spontaneously hypertensive rat model. They demonstrated that despite continuous and marked LV wall thickening, the LV eventually dilates and then fails. At this stage, the stimulus for LV hypertrophy is not only elevated arterial pressure but also chamber dilatation that further aggravates the hemodynamic load by increasing wall stress. This seminal finding laid the foundation for the concept that regardless of the initial insult, ventricular dilatation may become a self-sustaining process of deterioration in LV structure and function.
A consensus statement helped define remodeling as the “genomic expression resulting in molecular, cellular and interstitial changes that are manifested clinically as changes in size, shape and function of the heart after cardiac injury” [28]. Remodeling process is regulated by mechanical, genetic, and neurohormonal factors [29]. The importance of ventricular remodeling has increased with the observation that agents such as inhibitors of the sympathetic and renin-angiotensin-aldosterone systems that have beneficial effects in HF also generally attenuate or reverse ventricular remodeling [30–34], whereas agents that fail to improve clinical outcomes either have no effect on remodeling or have been associated with adverse remodeling [4]. Ventricular remodeling has therefore emerged as a credible surrogate end point and an important therapeutic target in HF [35, 36].
Mechanisms of Left Ventricular Remodeling
Although ventricular remodeling may occur following any form of myocardial injury [20], most of our knowledge has been acquired from the study of remodeling following MI. Acute coronary occlusion in the clinical setting or in the experimental animal leads to loss of myocardial tissue, depression of myocardial function, and hypotension. This causes baroreceptor-mediated activation of a number of neurohormones that help stabilize the hemodynamics through an increase in heart rate, contractility, and fluid retention. However, continuous activation of these mechanisms, designed for short-term support of blood pressure [37], may lead to progressive LV remodeling and dysfunction. Two distinct phases have been identified following MI: early postinfarct LV remodeling and late progressive LV remodeling.
Early Postinfarct Left Ventricular Remodeling
Loss of regional wall function after acute MI results in an abrupt increase in loading conditions of the ventricle that brings on a unique pattern of remodeling involving the infarct area, the border zone, and the remote noninfarcted myocardium. Thinning and stretching of the acutely infarcted myocardium lead to infarct expansion, the first feature of LV remodeling [21, 22]. Although later thinning of the LV wall also occurs in the noninfarcted myocardium, the cellular mechanisms are different in the two regions. In the infarcted myocardium, wall thinning is pronounced and is a result of loss of myocytes, collapse of the intercellular space, and stretching of surviving myocytes [21–23, 38, 39]. This may lead to bulging of the infarct zone that can result in ventricular rupture, aneurysm, mitral insufficiency, and ventricular tachyarrhythmias. In the noninfarcted regions, myocardium thins because of a decrease in the number of myocytes across the wall [38, 39]. Two mechanisms—myocyte slippage [38] and myocyte loss from necrosis [40–42] and apoptosis [41, 43]—have been proposed to explain this decrease.
It has been suggested that “myocyte slippage” plays a major role in progressive chamber dilation leading to failure [8, 38, 44], although much of the literature mentioning this phenomenon is rather vague. This concept usually refers to slippage of myocytes past one another transversely or linear slippage of individual myofibrils within myocytes [44, 45].
Increased myocardial collagenase activity (see discussion under Extracellular Matrix Remodeling below) is believed to disrupt intermyocyte collagen struts, leading to side-to-side slippage of myocytes [46]. Such a process could reduce wall thickness and increase the volume of the ventricle. Linzbach [44] and others [38] have noted reduced numbers of myocytes across the wall as evidence of myocyte slippage. However, this explanation may be too simplistic. For a meaningful discussion of the slippage concept, we need to consider the three-dimensional nature of myocyte-to-myocyte interconnections. Each myocyte is connected to an average of 5–10 neighboring myocytes via end-to-end and side-to-side intercalated disks (◘ Fig. 17.2) [47]. Slippage implies disruption of intercalated disks. Once the disks are disrupted, they may be unable to reconnect, resulting in poorly coordinated contractions.


Fig. 17.2
Scanning electron micrograph (top) and a drawing (bottom) of the cardiac myocardial fibers. The cardiocyte Ci connects with five neighboring cardiocytes (A 1, A 2, C 1, C 2, and C 3). Source: Yamamoto S, James TN, Sawada K, Okabe M, Kawamura K. Generation of new intercellular junctions between cardiocytes. A possible mechanism compensating for mechanical overload in the hypertrophied human adult myocardium. Circ Res. 1996;78:362–70
Factors Affecting the Magnitude of Remodeling after Myocardial Infarction
The magnitude of infarct expansion and development of LV remodeling largely depends on the extent of myocardial damage and the loading conditions of the ventricle. In the rat infarct model, increase in LV diastolic volume is related to the size of the infarct and correlated with the extent of impaired systolic performance [48, 49]. A critical transmural infarct size of about 20 % of LV myocardium was necessary for significant infarct expansion [22]. It was observed more frequently in patients with a large anterior transmural infarction compared to an infarction in other regions of the LV [50–52]. Distortion of the ventricular contour leading to aneurysm formation is frequent in patients with infarct expansion and is associated with a much higher 1-year mortality than for patients with anterior infarction and comparably reduced ejection fraction (EF) but without aneurysm [53].
The loading conditions of the ventricle are also important in ventricular remodeling. Both early transient increase in afterload after an MI and sustained increase in afterload with aortic banding increased infarct expansion in animal models [54, 55]. Patients with hypertension and LV hypertrophy have increased morbidity and mortality after MI [56], and careful afterload reduction early in the course of MI may have important effects on LV remodeling by reducing infarct expansion and limiting infarct size [57].
Early establishment of patency of the infarct-related coronary artery and restoration of antegrade flow may also confer a beneficial effect on ventricular remodeling and long-term survival in patients with acute MI, whether accomplished pharmacologically [58] or mechanically [59]. However, the open-artery hypothesis that the restoration of antegrade flow in the infarct-related artery days, weeks, or even several months after MI would improve survival with or without improvement of LV function was not proved in the Occluded Artery Trial [60] and the Total Occlusion Study of Canada (TOSCA)–2 Trial [61].
Late Progressive Postinfarct Left Ventricular Remodeling
Early infarct expansion after MI may be followed by progressive ventricular dilatation and dysfunction over subsequent months and years, involving predominantly the noninfarcted segments. The mechanisms responsible for this inexorable deterioration of LV structure and function are not entirely clear but are related to continued activation of neurohormones and cytokines such as norepinephrine, angiotensin II, aldosterone, endothelin, and tumor necrosis factor. These factors, in combination with increased wall stress and mechanical stretch of the myocytes, upregulate a large number of signaling pathways, leading to structural and functional changes in the myocyte and nonmyocyte compartments that underlay a reduction in LV function and the progression of HF. In the discussion that follows, changes in these individual components will be described and their implications discussed .
Alterations in the Myocyte Compartment
The remodeling process results in important changes in the cardiac myocytes. These include myocyte hypertrophy, myocyte loss by necrosis [41, 42, 62] and apoptosis [41, 63–66], and changes in the structural proteins with downregulation of contractile and sarcomeric skeleton proteins and upregulation of cytoskeletal and membrane-associated proteins [67]. In addition, loss of myofilaments, nuclear enlargement, development of multiple small mitochondria, decrease in the T-tubular system, and sarcoplasmic reticulum are common histological features of the failing myocardium [68].
Myocyte Hypertrophy
Grossman and coworkers proposed that alterations in myocyte shape and size determine the type of cardiac hypertrophy [69]. In conditions with pressure overload such as aortic stenosis or hypertension, parallel addition of sarcomere causes an increase in myocyte cross-sectional area with no increase in myocyte length (◘ Fig. 17.3) [70–72]. This leads to an increase in wall thickness and concentric LV hypertrophy (increase in ratio of wall thickness to chamber dimension) [69, 73]. In conditions with volume overload such as aortic and mitral regurgitation, ventricular volume and wall thickness increase proportionally, and this is associated with a corresponding proportional increase in both myocyte length and cross-sectional area (addition of sarcomeres both in parallel and series) [74] (◘ Fig. 17.3). It appears that during the compensated stage of concentric LV hypertrophy, wall stress does not increase.


Fig. 17.3
Schematic representation of myocyte change in left ventricular concentric and eccentric hypertension. In pressure-overload hypertrophy, myocyte cross-sectional area (CSA) increases and the ventricular wall becomes thicker during the compensatory phase. In volume-overload hypertrophy, ventricular volume and wall thickness increase proportionally, and this is associated with a corresponding proportional increase in both myocyte length and CSA. CSA and L, cross-sectional area and length. Source: Gerdes AM. The use of isolated myocytes to evaluate myocardial remodeling. Trends Cardiovasc Med. 1992;2(4):152–5
After a large MI, progressive LV dilatation is due to an increase in myocyte size which occurs predominantly by laying of sarcomeres in series, resulting in an increase in myocyte length, with only mild increase in width and cross-sectional area [75–78] (◘ Fig. 17.4). This further increases cavity volume with no change or a decrease in wall thickness. Myocyte length is the major determinant of changes in LV size, and most of the increase in LV volume can be explained by an increase in myocyte length [75, 77–79]. Although LV mass increases, the increase in LV volume is proportionately greater, so that mass-to-volume ratio, an important determinant of wall stress, is reduced. The development of myocardial hypertrophy after MI, therefore, results in eccentric hypertrophy (cavity dilation with a decrease in wall thickness to chamber dimension ratio) that increases wall stress.


Fig. 17.4
Cardiac myocyte remodeling in the rat infarct model. Myocyte length and width from rats at 2, 4, and 6 weeks after myocardial infarction are compared to those from a sham-operated animal. Note the predominant increase in myocyte length as the major determinant of the increase in ventricular volume. MI myocardial infarction. Source: Anand IS, Liu D, Chugh SS, Prahash AJ, Gupta S, John R, Popescu F, Chandrashekhar Y. Isolated myocyte contractile function is normal in postinfarct remodeled rat heart with systolic dysfunction. Circulation. 1997;96(11):3974–84
In volume-overload conditions such as mitral and aortic regurgitation, ventricular hypertrophy remains appropriate and helps to maintain normal wall stress for variable periods of time. Transition from a compensated to a decompensated state is associated with further increase in chamber volume but no increase in wall thickness. This results in a decrease in mass-to-volume ratio and increase in wall stress. The cellular mechanisms responsible for this are not entirely clear, but they could be related to an arrest in growth of the myocytes in the transverse diameter, resulting in myocyte lengthening without further change in myocyte cross-sectional area. Studies of mitral regurgitation in the dog, and in patients at the time of mitral valve surgery, also show a decrease in myocardial myosin content proportional to the degree of LV dysfunction [74, 80]. Thus, reduced contractility in mitral regurgitation could, in part, be due to loss of contractile elements. Although aortic and mitral regurgitation are often considered together as volume-overload conditions, the two have their specific pathophysiologic features .
In aortic regurgitation, the sum of the regurgitant and forward stroke volume is ejected into the aorta in systole, resulting in a wide pulse pressure and systolic hypertension. Therefore, aortic regurgitation creates both volume and pressure overload on the left ventricle. Systolic wall stress is always higher in aortic regurgitation than in mitral regurgitation [81] and is often as high as in aortic stenosis (the classic pressure-overload condition) [82]. These different loading conditions in mitral and aortic regurgitation create two different types of ventricular geometry. In mitral regurgitation, there is an enlarged thin-walled left ventricle in which the mass-to-volume ratio is less than 1.0 [83]. In contrast, in aortic regurgitation, the mass-to-volume ratio is normal at 1.0 [84]. Whether the cellular hypertrophy at the onset of failure is different in these two conditions remains to be determined.
In pressure-overload conditions, concentric ventricular hypertrophy (thick wall, normal chamber volume, and high mass-to-volume ratio) helps to keep wall stress normal despite high ventricular pressure. Because systolic stress (afterload) is a major determinant of ejection performance, the normalization of systolic stress helps to maintain a normal stroke volume despite the need to generate high levels of systolic pressure [12]. Transition to failure is accompanied by progressive cavity enlargement and decline in the mass-to-volume ratio, resulting in eccentric ventricular hypertrophy. In spontaneously hypertensive rats, transition to failure is preceded by myocyte lengthening without an increase in myocyte cross-sectional area [70, 71].
Myocyte Death
Cell death is an important determinant of progressive cardiac remodeling and LV wall thinning. A reduction of contractile material is a prominent feature in HF, and myocyte loss may occur either by necrosis or apoptosis [41].
Myocyte necrosis : Necrosis generally occurs in the setting of catastrophic events such as MI or inflammation and is characterized by severe membrane alterations, release of cell breakdown products, and polymorphonuclear infiltration. However, slow myocyte loss by necrosis is also a common feature of chronic HF [40–42, 85]. During the progression of HF, activation of several neurohormones occurs, including norepinephrine, angiotensin II, and endothelin. These neurohormones are directly toxic to the myocardium and have been shown to cause myocyte necrosis in various animal models [86, 87]. Moreover, in patients with severe HF, circulating levels of troponin are often increased, suggesting ongoing myocyte necrosis [88, 89]. Myocyte loss through necrosis probably contributes to progressive LV dilatation and wall thinning. Even very low plasma concentrations of troponin are predictive of adverse outcomes in patients with chronic HF [88].
Myocyte apoptosis : Apoptosis or programmed cell death is an evolutionarily conserved process of cell death, wherein cells die without provoking significant inflammatory response. Evidence shows that apoptosis contributes to the progression of HF. Apoptosis occurs through a cascade of subcellular events including cytochrome c release into the cytoplasm and activation of proteolytic caspases [90]. Activated caspases lead to fragmentation of cytoplasmic proteins, including contractile apparatus [91]. Caspase-3 (the final executioner in the apoptotic cascade) overexpression or activation has been shown to directly reduce the contractile performance of the LV [92]. The degree of myosin cleavage with caspases correlated with the contractile performance of the heart [93]. It has been proposed that the release of cytochrome c from mitochondria and contractile protein loss in living heart muscle cells contributes to systolic dysfunction [90]. Apoptosis is involved at multiple points in the natural history of HF. This includes initial events like ischemia, infarction, and inflammation as well as those events occurring later in established LV dysfunction. Several of the factors implicated in the pathogenesis of HF such as myocardial stretch [94], norepinephrine [95], angiotensin II [96, 97], tumor necrosis factor-α (TNF-α), and oxidative stress [98, 99] may provoke apoptosis.
While the presence of myocardial apoptosis has been confirmed in end-stage human HF [65, 66] and in several animal models [41, 43, 63, 64], questions remain whether apoptosis is a cause or a consequence of HF. Myocyte apoptosis may be a factor in the transition from compensated to uncompensated HF [91]. This has been shown in several animal models of experimentally induced LV hypertrophy and HF [100–102]. Several studies have demonstrated the presence of apoptosis late after MI [103–105].
Alterations in Myocyte Structural Proteins
The complexity of events involved in the pathogenesis of ventricular remodeling cannot be solely attributed to myocyte hypertrophy and cell loss. The hypertrophied myocytes in the remodeled failing heart also show alterations in most of the structural proteins (◘ Table 17.1) [67]. Following is a brief description of the structural protein (◘ Fig. 17.5), alterations that occur in HF proteins, and their functional consequences.

Table 17.1
Myocyte protein families
Contractile proteins | Myosin, α-actin, α-tropomyosin, troponins C, I, and T |
Sarcomeric skeleton | Titin, α-actinin, M-line proteins: M-protein, myosin-binding protein-C |
Cytoskeletal proteins | Tubulin, desmin, nonsarcomeric actin |
Membrane-associated proteins | Vinculin, talin, dystrophin, spectrin, integrins |
Proteins of the intercalated disk | Connexins, cadherins, catenins |

Fig. 17.5
Diagram of the myocyte sarcomeric proteins . (Courtesy of H. L. Granzier.) MyBP–C myosin-binding protein-C, Tn–C troponin C, Tn–I troponin I, Tn–T troponin T
Contractile Proteins
The contractile apparatus includes thick filament myosin and thin filament complexes composed of α-actin, α-tropomyosin, and troponins C, I, and T. Ventricular remodeling involves transcriptional and translational downregulation of these proteins [67]. One of the earliest changes is a decrease in α-myosin heavy chain and an increase in β-myosin heavy chain [106].
Sarcomeric Skeleton Proteins
The contractile apparatus is kept in register by different proteins localized in the Z-disk, M-band of the sarcomere, and the giant filament molecule titin, which spans the entire half-sarcomere from the Z-disk to the M-line. The Z-disk is a region of overlapping tails of actin microfilaments cross-linked by α-actinin. The M-line is the region where the myosin tails are linked and organized by the M-line proteins—myomesin, M-line protein, and myosin-binding protein-C. Titin is anchored with its N-terminus at the Z-disk and reaches the M-line region with its C-terminal head portion where it interacts with M-line protein and with myomesin [107]. It spans the Z-disk of the sarcomere [108] and overlaps in the M-line region of the sarcomere [109], thus functioning as a molecular spring and a source of elastic properties of the cardiomyocyte (◘ Fig. 17.5). The interplay between titin and actomyosin suggests a possible role for titin in the Frank-Starling mechanism of the heart [107]. Several studies have reported that the amount of titin is reduced in myocardium of patients with dilated cardiomyopathy, and this could be responsible for the altered ventricular compliance in this condition [110, 111]. Because titin is required for sarcomere formation, lack of titin may also contribute to contractile dysfunction of failing hearts [112].
Cytoskeletal Proteins
The cytoskeleton is a complex network of microtubules (primarily tubulin), nonsarcomeric actin, and intermediate filaments (primarily desmin). Tubulin is the protein of microtubules, which are hollow tubes formed from α- and β-tubulin surrounding the nucleus and spreading mostly in a longitudinal direction throughout the entire cell. The multifunctional roles of microtubules include mitosis, intracellular transport, organization of organelles, cell motility, determination of cell shape, receptor modulation, and signaling [113]. Desmin surrounds the Z-disks and connects the sarcomeres so that they are kept in register during contraction. Desmin filaments also link myofibrils to one another, to the sarcolemma, and to the nuclear envelope [114]. The desmin network plays a role in the underlying structural integrity of the myocyte, as well as participating in the signaling processes needed for integration of cellular responses to external and internal stimuli [114].
In failing human myocardium, both tubulin and desmin are increased [115]. The increase in these proteins mainly occurs in cells that lack myofilaments and could, therefore, help maintain cellular stability. Tubulin accumulation plays a role in certain models of pressure-overload hypertrophy [116]. In feline right ventricular hypertrophy resulting from pulmonary artery banding, isolated myocytes show contractile dysfunction and loss of compliance. These changes are accompanied by an increase in total and polymerized tubulin [117–119].
Desmin-related cardiomyopathies that have, as a hallmark, abnormal deposits of desmin aggregates are increasingly reported. A progressive increase of desmin protein and filaments was shown to accompany the transition from hypertrophy to HF [120]. Overexpression and altered distribution of desmin were also observed in dilated cardiomyopathy [115]. The absence of an intact desmin filament system may also be involved in cardiomyocyte hypertrophy and cardiac dilation with compromised systolic function [121]. Whether alteration in desmin quantity is a cause or a consequence of HF is not yet clear .
Membrane-Associated Proteins
Membrane-associated proteins include dystrophin, vinculin, talin, spectrin, and integrins, which are involved in fixation of sarcomeres to the lateral sarcolemma and stabilization of the T-tubular system [67, 122, 123]. Mutations of these proteins have been shown to cause dilated cardiomyopathy [124–126]. Dystrophin connects intracellular actin and extracellular laminin independent of integrin binding [127] and plays an important role in promoting the action of the cytoskeleton as a stabilizing force and as a mechanotransductor [128].
Intercalated Disk Proteins
The intercalated disk consists of three different types of specialized membranes: fascia adherens, desmosomes, and gap junctions [129]. Fascia adherens establish the longitudinal connections with the contractile filaments. The desmosomes are connected to intracellular desmin via desmoplakins. Connexins are four-pass transmembrane proteins that are assembled in groups of six to form hemichannels, or connexons, and two hemichannels combined to form a gap junction. Gap junctions are responsible for the orderly spread of electrical excitation from one myocyte to the next in the heart. Remodeling of gap junction and connexin expression is a conspicuous feature of human congestive HF and other cardiac conditions with a dysrhythmic tendency. Remodeling of gap junctions and reduced connexin43 levels may contribute to slowing of conduction [130, 131]. Evidence from experimental animals strengthens the case that gap junction remodeling is a key determinant of arrhythmias in the diseased heart [132, 133].
Alterations in the Nonmyocyte Compartment
Apart from the myocyte compartment, the chronically failing heart is characterized by iterations in the extracellular matrix (ECM), particularly by fibrous tissue formation [62]. Such an adverse accumulation of ECM raises myocardial stiffness and impairs contractile behavior [134].
Extracellular Matrix Remodeling
The extracellular matrix of the heart is made up of a number of structural proteins including fibrillar collagen, smaller amounts of elastin, laminin, fibronectin, and signaling peptides. The complex collagen three-dimensional weave, mainly consisting of type I collagen, interconnects individual myocytes through a collagen-integrin-cytoskeletal-myofibril arrangement. This network supports cardiac myocytes during contraction and relaxation and also provides a mechanism for translating individual myocyte shortening and force generation into ventricular contraction. It is also responsible for much of the ventricle’s passive diastolic stiffness [135]. In both human and animal studies, progressive LV remodeling and dysfunction are associated with significant changes in the ECM [136–139]. The specific changes in serological markers of collagen turnover occurring in HF with preserved versus reduced systolic function need to be clarified [140].
The structural hallmark of prolonged pressure-overload hypertrophy is increased collagen accumulation between individual myocytes and myocyte fascicles (◘ Fig. 17.6) [141, 142]. Thus, the highly organized architecture of the ECM undergoes significant alterations in collagen structure, composition, and geometry caused by increased collagen synthesis, postsynthetic processing, posttranslational modification, and decreased degradation and turnover. This “reactive” collagen deposition is characterized by both perivascular and interstitial fibrosis [135, 143, 144]. The changes in collagen homeostasis that occur during the development of chronic pressure-overload hypertrophy are directly associated with increased myocardial diastolic stiffness properties, which in turn cause abnormal diastolic filling [142, 145, 146]. Indeed, clinical evidence suggests that progressive ECM accumulation and diastolic dysfunction are important underlying pathophysiological mechanisms for HF in patients with pressure-overload hypertrophy [147, 148].


Fig. 17.6
Scanning electron micrographs taken from normal nonhuman primate left ventricular myocardium and following the induction of pressure-overload hypertrophy (POH). These microscopic studies demonstrate thickening of the collagen weave and overall increased relative content between myocytes with POH. Source: Abrahams C, Janicki JS, Weber KT. Myocardial hypertrophy in Macaca fascicularis. Structural remodeling of the collagen matrix. Lab Invest. 1987;56(6):676–83
Because of the persistently elevated preload in volume-overload hypertrophy, a much different pattern of ECM remodeling occurs. In large-animal models of volume-overload hypertrophy caused by chronic mitral regurgitation, the LV remodeling process is accompanied by increased degradation of collagen fibrils surrounding individual myocytes [80]. These changes in ECM support are associated with changes in isolated LV myocyte geometry where the cardiac cells increase in length. Representative scanning electron micrographs taken from a model of canine mitral regurgitation [149] are shown in ◘ Fig. 17.7 and show the profound differences in ECM structure and composition compared with normal myocardium. Increased ECM proteolytic activity likely contributes to the reduced ECM content and support and, thereby, facilitates the overall LV remodeling process [150].


Fig. 17.7
Scanning electron micrographs taken from normal canine left ventricular myocardium following chronic mitral regurgitation that causes a volume-overload hypertrophy (VOH). In this model of VOH, a loss of normal ECM architecture was demonstrated between individual myocytes (arrows), and the collagen supporting network is poorly organized. Source: Spinale FG. Myocardial matrix remodeling and the matrix metalloproteinases: influence on cardiac form and function. Physiol Rev. 2007;87(4): 1285–342
Although the mechanisms by which increased degradation of collagen promotes LV dilatation and global LV dysfunction are not entirely clear, dissolution of the collagen weave may lead to increased elasticity and contribute to muscle fiber slippage and, therefore, an increase in chamber size [145]. Loss of collagen struts connecting individual myocytes could prevent transduction of individual myocyte contractions into myocardial force development, resulting in reduced myocardial systolic performance.
The ECM and, particularly, collagen are under dynamic control of two sets of proteins: those that favor degradation and those that tend to inhibit it. The dissolution or degradation of collagen is predominantly related to the activation of matrix metalloproteinases (MMPs ), a family of zinc-containing proteins that includes collagenases, gelatinases, stromelysins, and membrane-type MMPs [150]. A critical control point for MMP activity is through the inhibition of the activated enzyme by the action of a group of specific MMP inhibitors termed tissue inhibitors of metalloproteinases (TIMPs ) [150]. The TIMPs are low-molecular-weight proteins that can combine noncovalently to active MMPs, inhibiting their activity [151, 152].
While the contributory mechanisms for the changes in plasma MMP levels remain speculative, an association between changes in plasma MMP levels to adverse LV remodeling has emerged. A Framingham Heart substudy showed that increased plasma MMP-9 levels were associated with LV dilation [153]. Elevated TIMP-1 plasma levels have been associated with major cardiovascular risk factors and with the presence of LV hypertrophy [153]. Furthermore, changes in plasma TIMP-1 levels have been associated with increased mortality [154]. However, it is likely that the changes in plasma MMP and TIMP levels observed in these studies will be influenced by the underlying etiology of the cardiovascular disease process and, therefore, that future studies will be needed. Furthermore, these studies only measured MMP and TIMP plasma levels at one point in time, so the temporal relation to the natural history of the LV remodeling process and progression to HF remains to be established.
Myocardial Fibrosis
Fibrosis in HF is an ongoing, active process of increasing collagen concentration and not simply a response to myocyte injury [134]. There are two types of fibrosis: reparative and reactive. Reparative fibrosis occurs in response to a loss of myocardial cells and is mainly interstitial. In contrast, reactive fibrosis is observed in the absence of cell loss as a reaction to changes in myocardial load or inflammation and is primarily perivascular. During ventricular remodeling, reactive and reparative fibrosis usually coexist. After MI, reparative fibrosis is organized as a scar and is surrounded by reactive fibrosis and myocyte hypertrophy [135].
The mechanisms responsible for fibrosis are still controversial. Fibrosis is not directly induced by myocardial stretch or mechanical overload. Chronic volume overload due to exercise training, atrial septal defect, or aortic insufficiency is not accompanied by ventricular fibrosis [155, 156]. In contrast, pressure overload is frequently associated with fibrosis. It has been proposed that ventricular fibrosis seen in arterial hypertension is caused by associated factors linked to this condition, such as ischemia [157] and neurohormones [134]. Humoral factors, particularly those of the renin-angiotensin-aldosterone system, are believed to be responsible for fibrosis. Angiotensin II and aldosterone have been implicated in the process as they stimulate collagen synthesis in cultured cardiac fibroblasts, and angiotensin II inhibits collagen degradation [158, 159].
Myocardial fibrosis has a number of deleterious effects on cardiac function. A two- to threefold increase in myocardial collagen content alters ventricular filling properties particularly by increasing diastolic stiffness; a fourfold or greater increase in fibrosis also affects systolic function [160]. Fibrosis contributes to ventricular arrhythmias because disproportionate collagen accumulation creates myocardial electrical heterogeneity. Fibrosis is therefore one of the major biological determinants of fatal issues in cardiac remodeling, including congestive HF, severe arrhythmias, and sudden death .
Changes in Global Structure and Function
The mechanical effects of LV remodeling set in motion several self-sustaining deleterious consequences. As the ventricle enlarges, LV geometry alters from a normal prolate ellipse to a mechanically disadvantageous spherical or globular shape. The result is an increase in meridional wall stress [161], abnormal distribution of fiber shortening, increase in oxygen consumption [161, 162], and abnormal myocardial bioenergetics [163]. The spherical shape of the LV leads to dilatation of the atrioventricular ring and stretching of the papillary muscles, resulting in functional mitral regurgitation [164], which contributes to a further decrease in forward cardiac output. Moreover, the high LV end-diastolic volume and pressure promote subendocardial ischemia that aggravates LV dysfunction and neurohormonal activation, decreases exercise capacity [165], and increases the risk of ventricular arrhythmias [166].
Compensatory Versus Maladaptive Remodeling
A fundamental question that must be addressed before embarking on a strategy to reverse hypertrophic and structural myocardial remodeling is whether remodeling is good or bad. Distinction is often made between a compensatory (adaptive) and a maladaptive process. An adaptive component enables the heart to maintain function in response to pressure or volume overloading in the acute phase of cardiac injury [167]. Acute distension of the viable myocardium and the operation of the Frank-Starling mechanism through an increase in sarcomere length are, therefore, entirely appropriate beneficial responses. Likewise, augmentation of chronotropic and inotropic activity through adrenergic receptor stimulation that tends to maintain pump function during the abrupt loss of contractile tissue can be considered compensatory.
Progressive LV dilatation after MI can also help to maintain stroke volume in the face of reduced contractile function and has been considered an adaptive and compensatory response [3, 168]. Under these circumstances, however, increased LV volume is not due to sarcomere stretch, but because of the addition of new sarcomeres in series [78]. Therefore, it is not a mechanism of enhancing contractility on the basis of Frank-Starling mechanism. Such a progressive remodeling and LV dilation does not normalize, but increases wall stress and is associated with a poor prognosis [19, 168].
Moreover, the prevention of very early LV dilation with the use of angiotensin-converting enzyme (ACE) inhibitors and beta-blockers does not have any deleterious hemodynamic consequence [169–171]. Indeed, Sharpe et al. [169] have demonstrated that the attenuation of LV remodeling with early initiation of ACE inhibitors is associated with a greater increase in stroke volume as compared with a placebo. Furthermore, the prevention of remodeling by early initiation of an ACE inhibitor or beta-blocker, after MI, in selected populations with LV dysfunction [31, 34] and even in unselected populations [172] is associated with significant reduction in mortality and morbidity [34, 170, 173, 174]. Hence, ventricular remodeling and dilation after MI may be maladaptive from the very start and should be a target for aggressive antiremodeling therapy .
In contrast, an increase in ventricular mass that helps to normalize wall stress in aortic stenosis and hypertension may be an appropriate compensatory response. Because systolic stress (afterload) is a major determinant of ejection performance, normalization of systolic stress helps to maintain a normal EF while generating high levels of systolic pressure [12]. However, LV hypertrophy has been shown to be an important independent risk factor for mortality and morbidity [17]. Similarly, a proportional increase in chamber volume, wall thickness, and mass in mitral and aortic regurgitation normalizes wall stress and is an obligatory response to maintain a large stroke volume that is necessitated by the regurgitant volume. Until the initial volume and pressure overload is matched by adequate hypertrophy, the process may be considered adaptive and compensatory. Eventually, a mismatch occurs with progressive dilation and the process becomes maladaptive and decompensatory, and HF becomes clinically manifest [175, 176]. There is no data to indicate when the transition from possible adaptive to maladaptive remodeling occurs; such a transition and its time course can vary greatly. However, once established beyond a certain phase, remodeling likely contributes to progression of HF. Thus, whether remodeling is beneficial or deleterious cannot be viewed as a stereotypical process. Today’s challenge is taking advantage of the adaptive features of the hypertrophic response while eliminating or at least minimizing the maladaptive consequences.
Reverse Remodeling
“Reverse remodeling” is a concept, where progressive LV dilatation and deterioration in contractile function are not simply arrested, but partially reversed. Two important questions related to reverse remodeling are: “Do myocytes have the ability to remove sarcomeres?” and “Is there any time line beyond which reverse remodeling cannot be achieved?”
Surgical and pharmacological experiments have confirmed that the regression of myocyte hypertrophy with removal of sarcomeres is possible. But insufficient data exist to address the second question. Remodeling is believed to be reversible early in the natural transition from hypertrophy to failure, whereas later, with the development of extensive fibrosis, accumulation of cytoskeletal proteins, and loss of the contractile filaments, an irreversible process sets in [111]. Several therapeutic approaches for HF have been shown to halt or even reverse the remodeling process.
Pharmacological Approaches
Numerous experimental studies have shown that modulating neurohormonal activation improves cardiac remodeling [177, 178]. McDonald et al. [179] showed that ACE inhibition and beta-adrenoreceptor blockade can reverse established ventricular remodeling in a canine model of discrete myocardial damage [179]. A significant reduction of LV mass and a trend in reduction of end-diastolic volume were found in both captopril- and beta-blocker-treated groups compared with the control group [179]. Tamura et al. [180] reported that the administration of angiotensin II type 1 receptor blockers produced significant reduction in myocyte volume, length, and cross-sectional area in rats with spontaneously hypertensive HF—below pretreatment values, suggesting true reverse remodeling, rather than simply arrested progression of myocyte hypertrophy [180]. Xu et al. [181] studied the effect of angiotensin II receptor blocker losartan combined with exercise training in a postinfarction rat model and demonstrated that exercise training after MI provides a beneficial effect on cardiac function and LV remodeling by altering the gene and protein expressions that regulate myocardial fibrosis. In contrast, such effects were only slightly improved by combining exercise and losartan [181].
ACE Inhibitors
The first class of medications shown to beneficially affect remodeling and clinical outcomes in patients with HF was the ACE inhibitors. In several trials performed in both asymptomatic and symptomatic patients with reduced EF, ACE inhibitors attenuated the progressive increase in end-diastolic and end-systolic volume compared with placebo-treated groups [32, 169, 182–184].
Beta-Blockers
In contrast to ACE inhibitors that attenuate LV remodeling, the use of beta-blockers has been associated with significant reduction in ventricular volumes and improvement in global LV function (reverse remodeling) [30, 31, 33, 185]. Beta-blockers were shown to reduce myocardial apoptosis which, at least in part, could explain their favorable effect on ventricular remodeling [186].
Aldosterone Receptor Blockers
Aldosterone receptor blockers have been shown to reverse LV remodeling following MI and in patients with HF [187, 188]. The 4E–Left Ventricular Hypertrophy Study [189] used cardiac magnetic resonance imaging (MRI) to compare LV mass regression by the selective aldosterone blocker eplerenone to the ACE inhibitor enalapril and the combination of eplerenone/enalapril in hypertensive patients with LV hypertrophy. Eplerenone was as effective as enalapril in regression of LV hypertrophy and control of blood pressure. The combination of eplerenone and enalapril was more effective in reducing LV mass and systolic blood pressure than eplerenone alone [189]. In a single-site clinical trial, Chan and colleagues [190] demonstrated with serial cardiac magnetic resonance (CMR) that the addition of spironolactone to candesartan has significant beneficial effects on LV reverse remodeling in patients with mild to moderate chronic systolic HF .
Angiotensin Receptor Blockers
Several trials demonstrated the beneficial effect of angiotensin receptor blockers (ARBs) on LV remodeling. In the Valsartan Heart Failure Trial (Val-HeFT) [191, 192], valsartan therapy attenuated LV remodeling [193]. Stratification by baseline severity of remodeling showed that patients with worse LV enlargement and systolic function are at highest risk for an event, yet appear to gain the most antiremodeling effect and clinical benefit with valsartan treatment [194]. The Losartan Intervention For Endpoint (LIFE) study [195] showed that reduction in LV mass by angiotensin II blockade was independent of blood pressure reduction, indicating that the inhibition of the renin-angiotensin-aldosterone system has added benefits beyond blood pressure control [195].
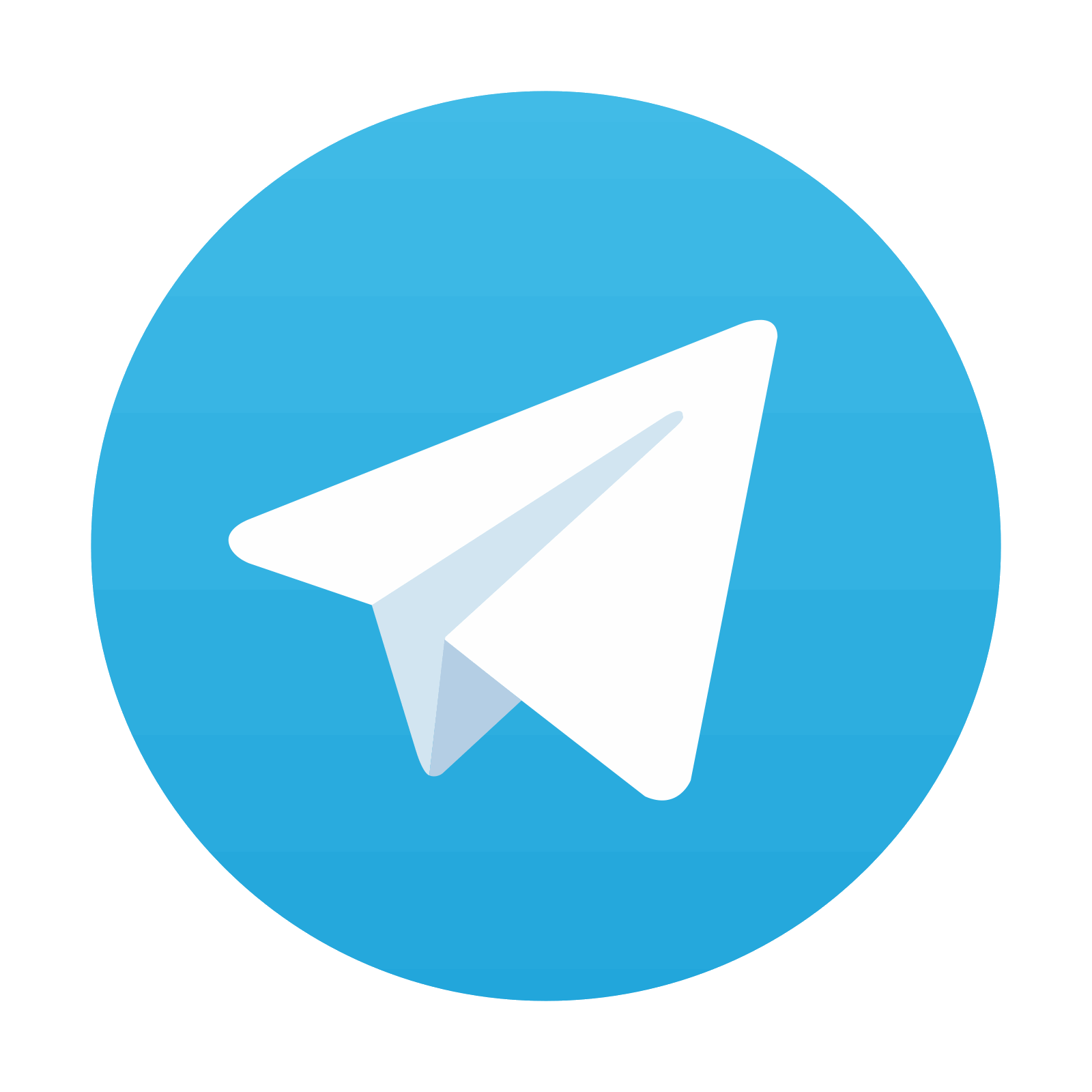
Stay updated, free articles. Join our Telegram channel
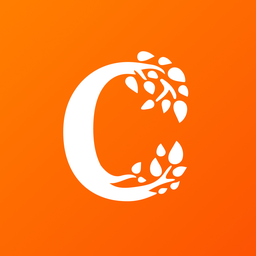
Full access? Get Clinical Tree
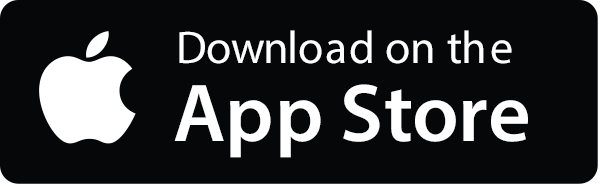
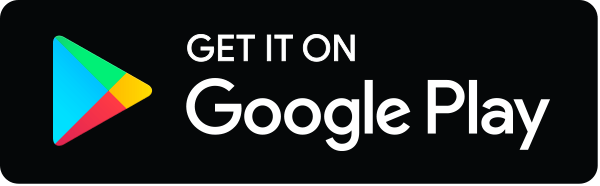