Dobutamine
Adenosine or regadenoson
Severe systemic arterial hypertension (≥220/120 mmHg)
Unstable angina pectoris
Significant aortic valve stenosis (peak aortic valve gradient >50 mmHg or aortic valve area <1 cm2)
Complex cardiac arrhythmias including uncontrolled atrial fibrillation
Hypertrophic obstructive cardiomyopathy
Myocarditis, endocarditis, pericarditis
Uncontrolled congestive heart failure
2nd or 3rd degree atrioventricular (AV) block or sinus node dysfunction
Systolic blood pressure less than 90 mmHg
Sinus bradycardia (heart rate <40 bpm)
Active bronchoconstrictive or bronchospastic disease with regular use of inhalers
Known hypersensitivity to adenosine or regadenoson
(Side effects are described as less significant with regadenoson than with adenosine, however, the half life of regadenoson is longer)
Atropine
Narrow-angle glaucoma
Myasthenia gravis
Obstructive uropathy
Obstructive gastrointestinal disorders
Stress Wall Motion Assessment
Stress wall motion assessment by CMR can be performed using pharmacological stressors (most commonly dobutamine) or physiological stress using bicycle or treadmill ergometers. Pharamcological stress is the more commonly used approach while physiological stress remains a research application.
Stress Modes
Ergometer Stress
Ergometer stress can be performed with either a (supine) scanner mounted bicycle ergometer or a treadmill near or in the scanner room. With supine cycling, it can be challenging to achieve target physiological responses. Treadmills offer a more physiological exercise regime but require rapid transfer of patients onto the scanner for imaging. Recently, an MR compatible treadmill has been described, which can be located immediately adjacent to the scanner with shortened transfer times [3].
Dobutamine Stress CMR
Dobutamine directly stimulates β1 and β2 receptors in the heart, leading to a dose-related increase in heart rate, blood pressure and myocardial contractility.
Dobutamine stress CMR testing is performed using standard protocols in which the dose of intravenous dobutamine is determined by the patient’s bodyweight. Dobutamine is administered in increments of 5–10 μg/kg/min to a maximum of 40 μg/kg/min until the target heart rate, calculated as ((220-age) × 0.85), is reached. If required to achieve the target heart rate, intravenous atropine may be added up to a maximal dose of 2 mg.
Because of the inability to use the electrocardiogram for ischemia detection, close monitoring of the patient is mandatory: ischemia detection relies on patient symptoms, physical signs and imaging findings. At each dobutamine stress level, a series of cine images is acquired and reviewed by the examiner immediately after data acquisition. The presence of new or worsening wall motion abnormalities is a termination criterion alongside other published criteria (Table 14.2) [4].
Table 14.2
Termination criteria for dobutamine and vasodilator stress CMR
Dobutamine stress CMR | Vasodilator stress CMR |
---|---|
Submaximal heart rate reached [(220–age) × 0.85] Blood pressure decrease >20 mmHg systolic below baseline systolic blood pressure or decrease >40 mmHg from a previous level Blood pressure increase >240/120 mmHg Intractable symptoms New or worsening wall motion abnormalities in at least 2 adjacent left ventricular segments (out of 17) Complex cardiac arrhythmias | Persistent or symptomatic AV block Significant drop in systolic blood pressure (>20 mmHg) Persistent or symptomatic hypotension Severe respiratory difficulty |
Vasodilator Stress
Vasodilator stress (see next section as well as the chapter on Perfusion for more details) can in principle be used for the induction of ischemic wall motion abnormalities, but has a significantly lower diagnostic accuracy for the detection of CAD [2]. The use of vasodilator stress to detect ischemic wall motion changes is not advised.
Pulse Sequences for Stress Wall Motion Assessment
Images are acquired in multiple planes (typically at least three short axis and two orthogonal long axis planes) at each stress level. See Fig. 14.1.
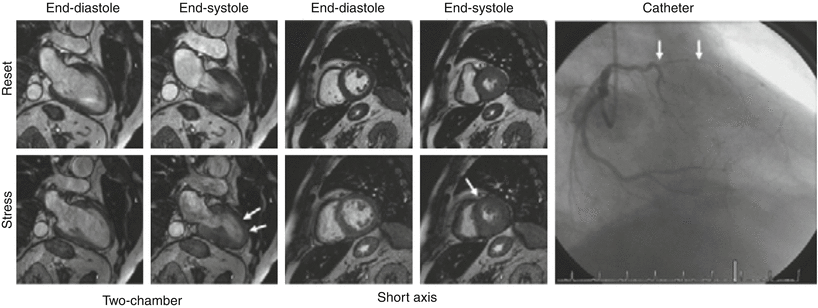
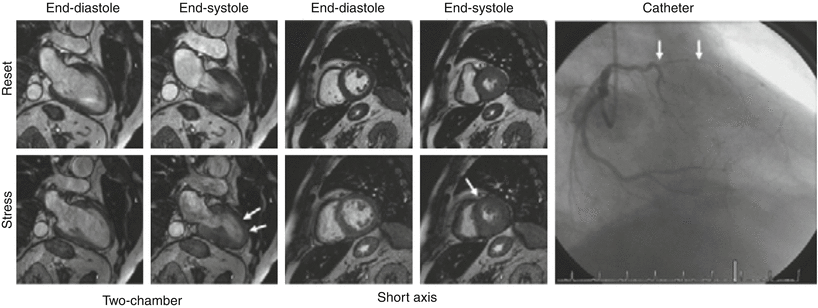
Fig. 14.1
Dobutamine-stress magnetic resonance in a patient with suspected CAD. At baseline: normal increase in systolic wall thickening shown in two-chamber and mid-short-axis views. During dobutamine stress, onset of hypokinesia in the anterior and antero-septal segment. Catheterization showed double-vessel CAD with high-grade stenosis of the left anterior descending artery was found (white arrows) (From Kelle et al. [42])
The CMR methods used for stress wall motion imaging are similar to those used for standard cine imaging. Typically retrospectively gated balanced steady state free precession (bSSFP) cine pulse sequences are used. Adjustments to the standard pulse sequences may be made to allow for higher heart rates and reduced breath-hold capacity during stress testing. The aim of pulse sequence design is to achieve a breath hold duration of 4–6 s, a temporal resolution of >25 phases/cardiac cycle and in-plane spatial resolution of 1.5–2 × 1.5–2 mm with a slice thickness of 8–10 mm [5]. To achieve these requirements the following adjustments are often made:
Use of (higher factor) parallel imaging (so that fewer lines of k-space need to be acquired, reducing acquisition time)
Reduced temporal resolution (so that more lines of k-space can be acquired in each R-R interval for each cardiac phase)
Reduced spatial resolution (use of a smaller matrix at similar field of view, resulting in fewer required lines of k-space)
Many other adjustments can be made and with a combination of these, real time cine acquisition and monitoring can be achieved [6].
Making Good Images: Practical Considerations
The main challenge in stress wall motion CMR is the acquisition of artifact-free images at the high heart rates encountered in patients that may be less able to breath-hold. As outlined above, pulse sequences used in stress cine imaging need to be set up to allow for these requirements.
Generally, fast gradient recalled echo (FGRE) imaging enables much faster data acquisition than spin echo imaging and is thus used in dobutamine stress protocols. However, in spoiled non-balanced FGRE acquisitions, the image contrast is heavily dependent on flow effects, with reduced signal and contrast between the wall and blood particularly in segments with impaired wall motion or globally in patients with heart failure. These limitations can impact image quality particularly at 3 T and at high heart rates achieved with medium and high dosages of dobutamine.
On current scanners, bSSFP sequences have therefore replaced FGRE cines. bSSFP provide a higher signal to noise ratio, do not suffer from flow related artifacts enabling a good contrast between the blood and the myocardium. The elevated signal to noise ratio typical for bSSFP allows the use of parallel imaging with higher acceleration factors, resulting in high speed and high contrast acquisitions and in a significant reduction of breathing artifacts, particularly if the images are acquired in the end-expiratory phase. These properties make bSSFP cine imaging particularly useful during stress testing. bSSFP sequences are however very sensitive to off-resonance artifacts in particular at higher field strength and with rapid blood flow, for example in the descending aorta, can result in image artifacts, particularly in the four chamber view. Pulsatile flow creates ghost artifacts of the vessel extending across the image in the phase-encoding direction. Modifying the phase-encoding direction and more accurate shimming can reduce significantly the occurrence and extent of these artifacts.
At high heart rates, VCG artifacts due to magneto-hydrodynamic effects are relatively common, leading to poorer image quality and sometimes resulting in non-diagnostic scans. Their occurrence can be minimized by careful preparation of the patient to ensure good electrical contact of the VCG electrodes. Moreover, different geometric schemes can be used for positioning the electrodes in order to maximize the signal.
In patients with MR conditional metallic implants, FGRE sequences sometimes enable superior image quality in comparison to bSSFP, which are more susceptible to inhomogeneities of the magnetic field.
The ideal cine approach for dobutamine stress CMR may be real-time techniques, considering the often unpleasant sensation of having a positive inotropic and chrontropic drug infused while laying still. Real-time cine imaging, while not necessarily the most suitable approach for ventricular function quantification, is more than adequate (and still usually superior to echocardiography-based cine imaging with dobutamine stress echo) for visual assessment of regional wall motion at rest and at each stage of dobutamine infusion. Real-time cine vs. segmented cine imaging eliminates not only the need for breath-holding but also a regular cardiac rhythm, recognizing that ectopic heart beats are more likely to occur with higher doses of dobutamine in many cardiac patients.
Analysis
For image analysis of stress wall motion studies, the myocardium is divided into 17 segments as defined by the American Heart Association [7]. Each of these segments is visualized in two standard views (apical, mid and basal short axis view, four-chamber, two-chamber and three-chamber view). Images acquired at rest and at incremental dobutamine dose levels should be displayed simultaneously and their display should be synchronized. Worsening or lack of improving wall motion or systolic wall thickening is considered a sign of ischemia. Segmental wall motion can be graded on a four-point scale (normokinetic, hypokinetic, akinetic, dyskinetic) and an overall wall motion score generated as the sum of segmental scores divided by the number of analyzed segments. Newer methods such as feature tracking may have a role for more objective and partly automated wall motion analysis form cine images.
Safety of Dobutamine-Stress CMR
The induction of ischemia has inherent risks, most prominently the risk of inducing malignant tachyarrhythmias. However, if safety considerations are addressed, dobutamine stress CMR is a safe procedure. In a series of 1,000 consecutive patients undergoing dobutamine-stress CMR, one sustained ventricular tachycardia was encountered and no cases of death or myocardial infarction occurred [8]. These data are similar to dobutamine stress echocardiography.
Diagnostic Performance of Wall Motion-Stress CMR
Several single-center studies have reported on the diagnostic performance of wall motion-stress CMR in patients with suspected CAD. These were summarised in a meta-analysis that showed a sensitivity of 83 % with a specificity of 86 % for all stressors (dobutamine, exercise or dipyridamole) [9]. Multi-center evidence is not available. Several studies have also suggested that stress-CMR is superior to stress echocardiography due to better image quality and unlimited imaging windows, but most of these studies preceded the introduction of the current standard of second harmonic echocardiography and other improvements in echocardiographic image quality [10]. Diagnostic accuracy and prognostic value of CMR stress wall motion testing may be improved by the use of strain imaging (e.g. SENC) or feature tracking [11, 12].
Prognostic Value of Dobutamine-Stress CMR
The prognostic value of dobutamine-stress CMR, but not that with other stressors, has been reported in several single center studies. All of these studies have shown a low cardiac event rate in patients with negative dobutamine-CMR testing of 1–2 % per year, with much higher event rates in patients with positive CMR studies. The largest cohort by Gebker et al. reported on 1,532 patients examined with dobutamine-CMR and found a 1.5 % death rate in patients with a negative test while patients with a positive test had a 6 % death rate [13].
Functional Assessment of Viable Myocardium
Dobutamine-CMR also allows an assessment of the functional reserve and thus viability of myocardium. In the context of chronic ischemia, myocardium may be hibernating, meaning that its metabolic and functional activity is down regulated. In the setting of acute myocardial infarction, ‘stunning’ can have similar effects, with reduced or absent contraction despite viable myocardium being present. With low-dose (5–10 mcg/kg/min) dobutamine stimulation, hibernating myocardium can be stimulated and shows an improvement of contractile function. With further increases of dobutamine dose, the flow limitation of an upstream coronary stenosis leads to ischemia and a reduction in contractile function, provoking the typical “biphasic response” of resting a wall motion abnormality, improvement at low dose, and deterioration at high dose dobutamine. Such functional assessment may be complementary or even superior to LGE imaging [14]. Serial cine CMR with doses of dobutamine may be particularly appealing as an alternate approach to LGE to assess myocardial viability in circumstances such as advanced renal insufficiency where administration of gadolinium-based contrast is contraindicated.
Myocardial Perfusion CMR
Basic Principles
In myocardial perfusion CMR, a bolus of an MRI contrast agent is injected into a peripheral, usually antecubital vein with an automated power injector, followed by a flush of saline. A dynamic series of images is acquired (typically for 40–60 heat beats) to track the myocardial passage of the contrast agent bolus. Figure 14.2 illustrates this principle.
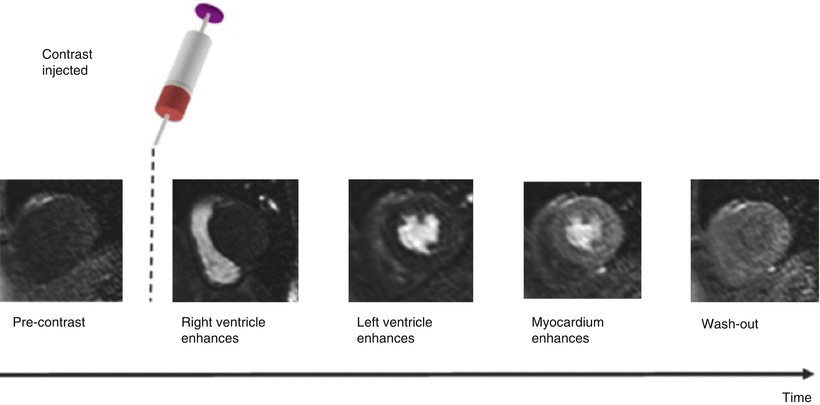
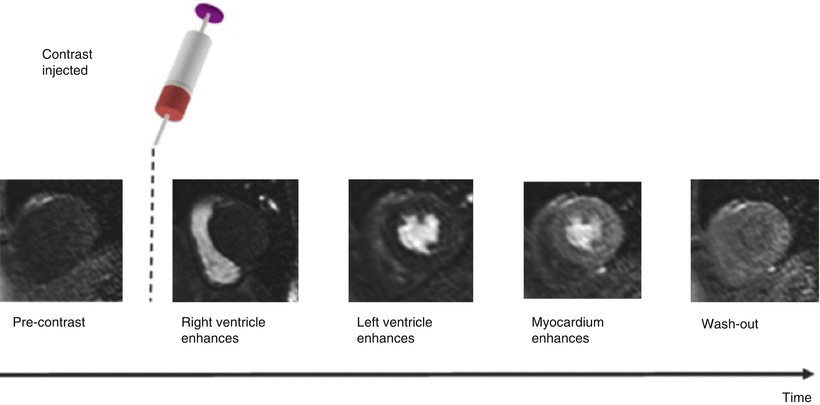
Fig. 14.2
Contrast agent is injected whilst the dynamic scan is in progress. Contrast agent can be seen as signal enhancement in the right ventricle (RV) followed by the left ventricle (LV) and more gradually in the myocardium, before finally washing out (From Biglands et al. [15] http://creativecommons.org/licenses/by/2.0/legalcode)
Image acquisition is generally performed at rest and during stress, most commonly applied as pharmacological vasodilator stress using adenosine, dipyridamole or regadenoson. All of these agents aim to induce maximal myocardial hyperemia through direct or indirect stimulation of the coronary adenosine receptors. Myocardium supplied by significantly stenosed coronary arteries has reduced perfusion reserve and thus takes up less contrast agent and appears darker compared with remote myocardium. Alternatively, inotropic or physiological stress can be applied as described in Sect. 3 and perfusion imaging performed at peak stress. In this case, the images depict myocardial ischemia directly rather than MBF reserve. Figure 14.3 shows an example of such an inducible perfusion defect.
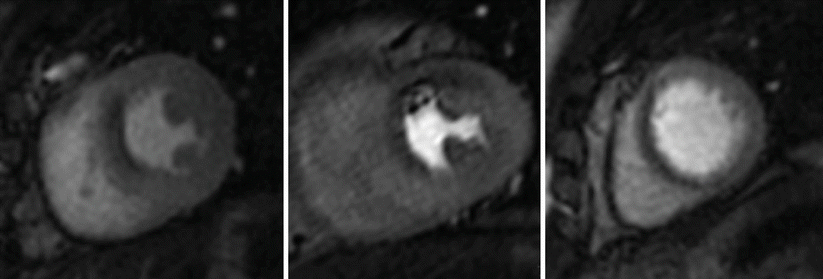
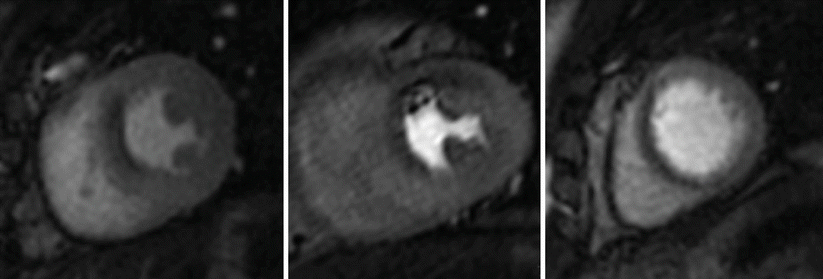
Fig. 14.3
Single dynamic image at peak myocardial contrast enhancement in a patients with a severe stenosis in the left anterior descending coronary artery. Inducible perfusion defect in the anterior and antero-septal segments (arrows). Note the lower signal intensity in these regions compared to the rest of the myocardium. Note also that the high in plane spatial resolution allows an assessment of the transmural extent of the perfusion defect
Stress Agents
Adenosine, dipyridamole and regadenoson are the most widely available vasodilators for pharmacologic stress testing. All of these agents dilate coronary vessels and can increase myocardial blood flow in myocardium subtended by unobstructed coronary arteries by 3.5 to 4 fold. Tissue supplied by stenotic vessels has reduced coronary flow reserve and thus shows an attenuated hyperemic response to pharmacological vasodilatation. Consequently, a differential perfusion reserve is seen in normal versus abnormally perfused myocardium, with the difference dependent on stenosis severity, absolute MBF and coronary collateralization. Vasodilators do not usually induce ischemia as all vascular beds are dilated through the medication, but in certain physiological settings (collateral-dependent circulation), coronary steal phenomena may induce regional ischemia.
Adenosine
Adenosine induces direct coronary arteriolar vasodilation through activation of the A2A receptor, but it is also activates A1, A2b, and A3 receptors, which leads to the side effects of adenosine infusion such as atrio-vertricular block, peripheral vasodilation (causing flushing sensation) and bronchospasm (causing chest discomfort). A modest increase in heart rate and often a small drop in systemic blood pressure are also observed. Adenosine is administered as a continuous intravenous infusion at a standard dose of 140 mcg/kg/min. Maximal hyperemia is typically reached at 3 min of infusion and image acquisition can begin. In the absence of any of these signs and symptoms, many investigators now recommend increasing the adenosine dose to 170 or 210 mcg/kg/min to overcome an assumed failure of full adenosine effect. Such as failure may be mediated by caffeine, which is a competitive antagonist of the adenosine receptor, if patients have failed to adhere to the recommended scan preparation.
Dipyridamole
Dipyridamole is an indirect coronary vasodilator that increases intravascular adenosine levels through inhibition of intracellular reuptake and deamination of adenosine. It is administered intravenously at a dose of 0.56 mg/kg over a 4-min period and induces hyperemia for more than 15 min.
Regadenoson
The more recently approved regadenoson is a selective A2A adenosine receptor agonist although it also has some effect on the A1, A2B and A3 adenosine receptors. Regadenoson is injected as a rapid (10 s) intravenous bolus of 5 mL (0.4 mg), followed by a saline flush. Regadenoson reaches its maximal effect within 1–4 min. In CMR, regadenoson stress imaging therefore commences at approximately 2–4 min. A key advantage of regadenoson over adenosine is the upfront bolus injection, so that only one cannula is needed, and a better side effect profile through more selective A2A receptor affinity. For example, first degree AV block occurs in 3 % with regadenoson (7 % with adenosine) and second degree AV block in 0.1 % (1 % with adenosine). It does however lead to a higher increase in heart rate (more than 40 bpm in 5 % vs 3 % for adenosine), which in some cases may result in reduced image quality.
Dobutamine
Dobutamine increases regional myocardial blood flow in response to its positive inotropic and chronotropic stimulation, which lead to increased oxygen demand. Vascular beds supplied by significantly stenosed coronary arteries have a reduced perfusion reserve and when oxygen demand exceeds supply, hypoxia and ischemia ensues, demonstrated on perfusion imaging as an area of reduced contrast uptake. Perfusion CMR during dobutamine stress faces challenges of high heart rates and higher risk profile than vasodilator imaging.
Physiological Stress
Physiological stress with an ergometer or treadmill can in principle also be used in perfusion CMR, although remains a research application.
Pulse Sequences for Myocardial Perfusion CMR: Challenges and Requirements
The key challenges in designing a pulse sequence for myocardial perfusion CMR are:
1.
Data must have strong T1 weighting to be sensitive to the signal intensity change induced by the MRI contrast agent.
2.
The dynamic contrast passage leads to different signal in each image, so that segmented multi-shot k-space filling is not feasible and single shot methods need to be used. Furthermore, at least three slices should be acquired at each heart beat with an in plane spatial resolution of at least 3 mm and at heart rates that may exceed 100 bpm during stress. Rapid data acquisition is therefore essential.
T1-Weighting
Signal enhancement of MRI contrast agents is predominantly the result of their effect on the T1 relaxation times of their surrounding tissues. Myocardial perfusion CMR pulse sequences therefore need to be strongly T1-weighted. Given the short TR required for fast data acquisition, T1-weighting in perfusion CMR is most commonly achieved with saturation recovery methods, i.e. by applying a 90-degree preparation pulse prior to the read-out pulse sequence. Saturation recovery methods are robust to heart rate variation, which is the reason why they have replaced previously used inversion recovery methods. The saturation time (TS) needs to be long enough to establish a high T1-contrast before the read-out sequence is employed and in most clinically used pulse sequences is around 100 ms. As for other MRI applications, the relationship between contrast agent concentration and signal is not linear, in particular at higher contrast doses and with longer TS. Shorter TS values and contrast agent doses may therefore be desirable if quantitative perfusion imaging analysis is planned – rarely the case in current clinical practice. Shorter TS also allows longer read out, usually allowing the acquisition of images with higher in-plane resolution, or acquisition of more slices within the constraints of each RR interval.
For signal readout, a wide variety of methods is in use, often vendor and institution specific. Most commonly, fast spoiled gradient echo methods (GRE), echo planar imaging (EPI), balanced Steady State Free Precession (bSSFP) pulse sequences or hybrids of these methods are used. Often, these readout schemes are combined with parallel acquisition techniques or image undersampling to shorten acquisition times and improve spatial resolution.
See Fig. 14.4 for typical myocardial perfusion CMR pulse sequences.
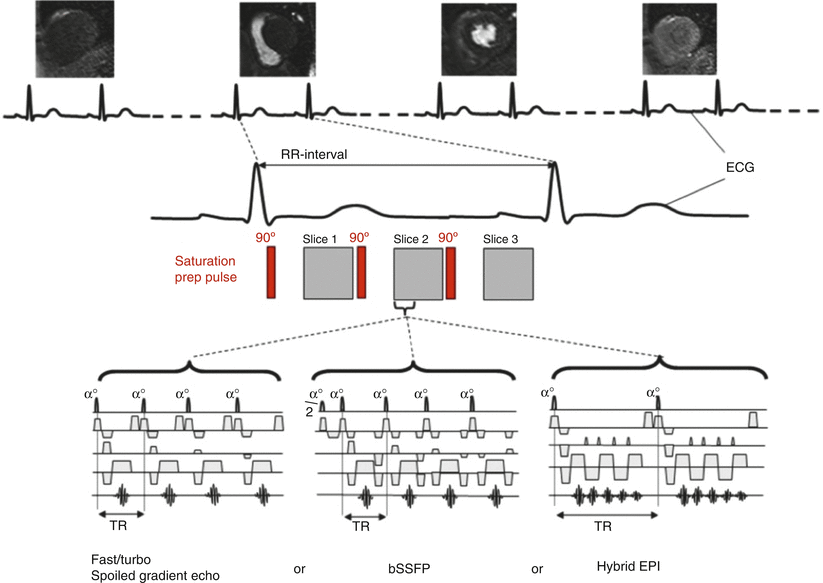
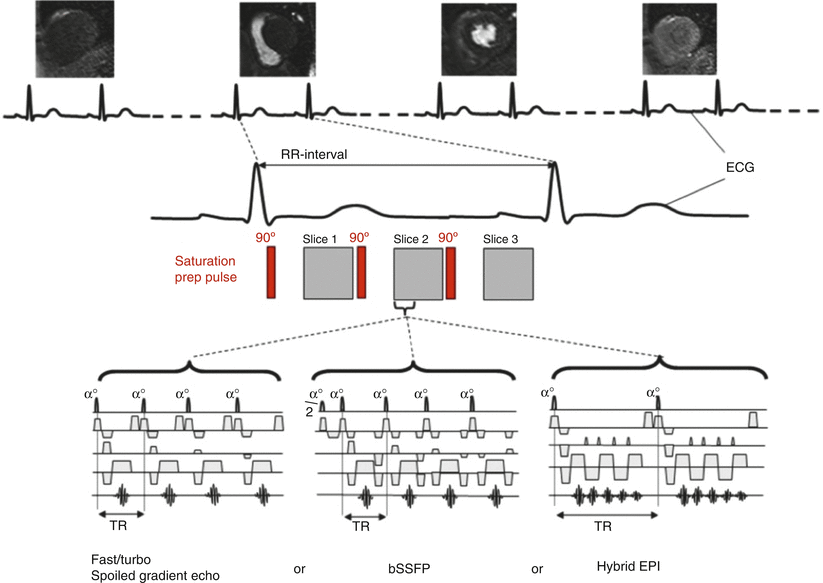
Fig. 14.4
Choice of imaging pulse sequence for myocardial perfusion MRI. Diagram illustrating the choices of data acquisition pulse sequence for myocardial perfusion MRI. A saturation recovery preparation pulse is employed to ensure T1-weighting followed after a delay by the image data acquisition pulse sequence. The most common data acquisition pulse sequences used are fast/turbo spoiled gradient echo (FGRE), balanced steady state free precession (bSSFP) or hybrid (or segmented) EPI. The number of slices that can be acquired (in this case three) is limited by the R-R interval, the saturation delay and the length of data acquisition period (From Biglands et al. [15] http://creativecommons.org/licenses/by/2.0/legalcode)
Fast Spoiled Gradient Echo (FGRE)
A fast gradient echo (FGRE) read-out sequence utilises a very short repetition time TR (<10 ms) and echo time TE (<5 ms), combined with a small flip angle of around 20–30°, resulting in a pulse sequence with predominant T1-weighting. A 90° preparation-pulse applied before the FGRE read-out increases image contrast. Since a very short TR is used, myocardial tissue or blood that stays in the slice becomes saturated (spoiling effect). Signal in FGRE therefore depends on the flow of blood to generate contrast. In clinical practice, FGRE pulse sequences are attractive because they are relatively robust to dark rim artifact.
Echo Planar Imaging (EPI)
This technique generates multiple gradient echoes following one rf pulse by quickly alternating the direction of frequency encoding gradients forming an echo train. The successive frequency gradients are interspersed by small phase encoding gradients to fill different lines of k-space within each heartbeat. However, T2* decay throughout the echo train causes image degredation with long echo trains. Thus a hybrid-EPI (also known as segmented EPI) technique is generally used in perfusion imaging where a number of shorter echo trains are acquired by applying multiple rf pulses. This reduces the destructive effect of T2* weighting, improving the image quality while maintaining part of the acceleration advantage provided by the EPI technique.
Balanced Steady State Free Precession
Balanced Steady State Free Precession (bSSFP) gradient echo sequences are constructed to protect the transverse magnetization from being spoiled but returned into phase at the end of each TR period when the next rf pulse is applied. Thus, the bSSFP sequence reverses the signal de-phased by the applied gradients by means of additional balancing gradients to re-phase the MR signal before each subsequent rf pulse. After a number of repetitions this gives rise to a steady state condition where the transverse magnetization from two or more successive repetition periods incorporate to give a much greater signal. Since the transverse magnetization is generated from multiple TR combination, the magnetization signal amplitude and consequently signal to noise ratio (SNR) for bSSFP is much higher in comparison to spoiled gradient echo. The increased signal permits the employment of higher receiver bandwidths, resulting in a shorter TE and TR compared to spoiled gradient echo pulse sequences and therefore improved imaging efficiency. However, if the magnetic field is not homogenous, the transverse magnetization from several TRs can destroy each other rather than sum together in areas of magnetic field inhomogeneity, causing a higher susceptibility to dark banding artifacts in comparison to spoiled FGRE. Shimming and keeping TR as short as possible are therefore crucial when bSSFP is used for myocardial perfusion CMR. Unlike spoiled gradient echo sequences, the contrast nature of bSSFP sequences is related to the tissue’s T2/T1 ratio, therefore fluid and fat in particular appearing as brighter than other tissues.
< div class='tao-gold-member'>
Only gold members can continue reading. Log In or Register a > to continue
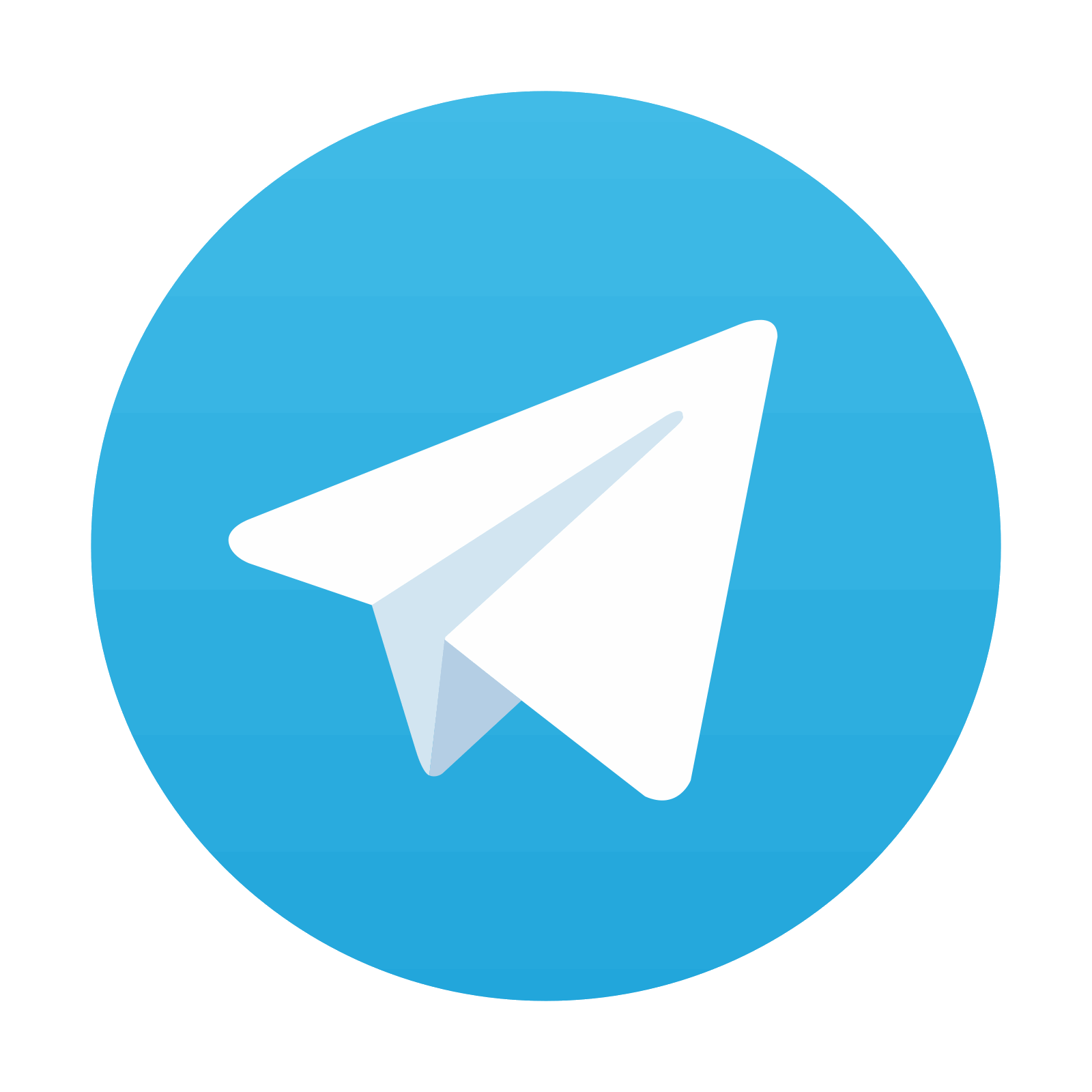
Stay updated, free articles. Join our Telegram channel
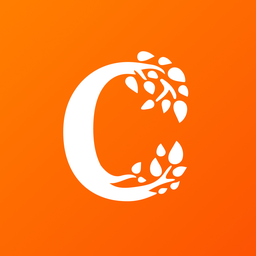
Full access? Get Clinical Tree
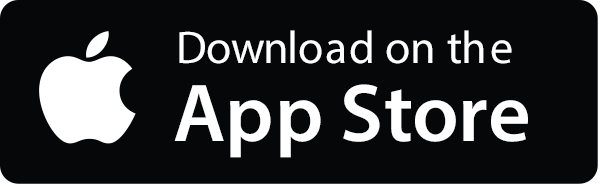
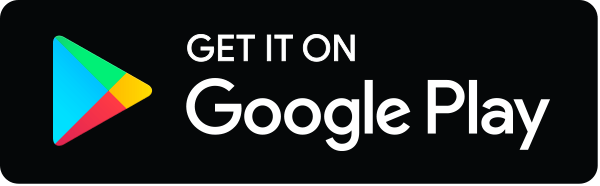