Fig. 14.1
HPA axis is the principal endocrine axis regulating the stress response. Stressors of different origins activate the hypothalamic CRH, which stimulates pituitary ACTH secretion and finally release of glucocorticoid from the adrenal gland. The HPA axis operates via forward positive feedback system, while the end-product, glucocorticoid exerts negative feedback regulation of CRH and ACTH. In addition to its expression in the central nervous system, several functions in peripheral sites have been identified. Genetic ablation of CRH (Crh knockout, Crh−/− mouse) in the heart negatively affects cardiac function, through the Erk1/2, Akt and NFκB signaling pathways
14.3.4.1 Glucocorticoid
Cardiomyocytes participate actively in the adaptive stress response via initiating a cascade of pathways to secure homeostasis. Failure to do so, results in extensive tissue damage, disease development and eventually HF. Glucocorticoid act by synchronizing the metabolic, autonomic, psychological, hemostatic and cardiovascular components of the stress response via its multiple tissue-specific genomic and non-genomic effects. These actions facilitate the vascular and metabolic effects of other stress hormones, such as catecholamines, glucagon and angiotensin-II, through stimulation of α1 adrenergic and angiotensin II receptor expression, and increase in the affinity and binding capacity of β-adrenergic receptors. In parallel, glucocorticoid suppresses associated processes such as inflammation, cellular proliferation and tissue repair processes. Finally, glucocorticoid prepare the organism for prolonged nutritional deprivation by facilitating proteolysis and support development of insulin resistance at the muscle level, while inducing gluconeogenesis and lipolysis. This pleiotropic action of glucocorticoid may be harmful for the cardiovascular system because of increased blood pressure and insulin resistance. Corticosteroid excess is associated with adverse cardiovascular outcomes. Patients with Cushings’s syndrome and primary aldosteronism, two conditions characterized by glucocorticoid excess and inappropriately high aldosterone production respectively, have increased risk for CVD. Prior to treatment of Cushing’s syndrome, it has not been uncommon for patients to experience early death from MI or stroke. In animal models, excess glucocorticoid can induce ATH. In humans, it has been suggested that corticosteroid-treated patients with rheumatoid arthritis and lupus erythematosus develop significantly more ATH than those not treated with steroids and the risk of ATH is related to the cumulative dose of corticosteroid. There have also been cases of adverse outcomes of steroid-dependent rheumatoid arthritis patients treated with thrombolytic agents for acute MI, possibly due to increased risk of myocardial rupture. However, there has been no consensus on the proposed adverse effects of glucocorticoid given acutely in MI [61].
On the other hand, glucocorticoid deficiency may result in hypotension, weight loss, hypoglycemia and death, particularly during stress; while glucocorticoid excess can lead to hypertension, insulin resistance, hyperglycemia and weight gain [62–64]. The vascular effects of glucocorticoid seem to be achieved by enhancing adrenergic stimulation, angiotensin II and possibly endothelin-1. Glucocorticoid upregulate angiotensin II type I receptor expression and α1 adrenergic receptors in rat vascular smooth muscle cells and potentiate the vasoconstrictive actions of angiotensin-II and norepinephrine in animals [65]
14.3.4.2 Crh and CRH-Related
In addition to its expression in the central nervous system, CRH has been identified in peripheral sites with functions not completely elucidated yet. So far, a dual, both anti- and pro- proinflammatory role for peripheral CRH has been identified. Its significant expression in inflamed human and rodent tissues due to the corresponding neutrophil and polymorphonuclear cell accumulation has been shown [66], and its effects have been confirmed in transgenic animal models [67–70]. Angiogenic effects of CRH, both in vivo and in vitro, have been shown [71, 72].
CRH belongs to an extended family of CRH-related peptides, which includes urocortins (UCN) (I, II and III). These peptides share significant homology with CRH, while they all bind the same family of receptors (CRHR1 and CRHR2), with CRH and UCN I binding both receptors, although with different affinities, and UCN II and III binding CRHR2 exclusively. UCNs and CRHRs are also widely expressed in a variety of tissues. CRH receptors belong to the G-protein coupled receptors (GPCRs) family and upon activation stimulate various intracellular pathways, in a time- and tissue-dependent manner. Expression of both CRHRs and UCN have been identified in the endothelium and the heart with CRHR2 robustly [73] and CRHR1 minimally if at all expressed [74]. Activation of phosphoinositide-3-kinase (PI3K)/Akt (protein kinase B/Akt) and mitogen-activated protein kinases (MAPK) in endothelial cells by CRHR2 links its expression with specific biological effects, while protective effects in cardiomyocytes during experimental ischemia have been shown [75, 76]. Furthermore we have recently found stimulation of VEGF by CRH via CRHR2 in endothelial cells [72, 77].
Cardioprotective effects of UCNs have been well documented in several experimental systems. In general, urocortins exhibit potent vasodilatory effects in arteries of different species through regulation of intracellular Ca2+ levels, increase heart contractility and evoke positive inotropic and lusitropic effects. More specifically, UCNs protect the heart from ischemia and reperfusion injury via improvement of post-ischemic cardiac performance, such as cardiac contractility, prevention from Ca2+ overload and reduction of cardiac cell death. These effects involve the activation of several signaling pathways targeting both cytoplasmic and mitochondrial processes. In particular UCN1, by initially binding to GPCRs, initiates the activation of PI3K, protein kinase A (PKA), Akt, protein kinase C (PKC) and MAPKs. Activation of these kinase pathways alters the activity of various channels including the mitochondrial permeability transition pore (MPTP), which is involved in the induction of cell death [78, 79], as described in Sects. 15.2.1 and 15.2.2. Apart from their acute effects, UCNs exert more prolonged, modulatory actions, such as transcriptional and translational effects on mitochondrial ATP-sensitive potassium channel (KATP), calcium insensitive phospholipase A2 (iPLA2) and protein kinase C epsilon (PKCε), all three involved in cardioprotection [80–84]. UCN1 acts via a p42/p44 MAPK (Erk1/2)-dependent signaling pathway in protecting both in vitro primary cell models and ex vivo and in vivo rodent heart from reperfusion injury. Erk1/2 phosphorylation and activation is mediated by the MAP kinase MEK1/2 following UCN1 treatment; while its inhibition disrupts UCN1-mediated cardioprotection [85, 86]. PI3K and its downstream effector Akt are crucial for UCN-stimulated increase in survival of cardiomyocytes [87]. Both MEK1/2 and PI3K are responsible for preventing pro-caspases -9 and -3 from being cleaved into their active forms [88, 89]. UCN2 and UCN3 also act through the PI3K pathway [75]. In addition to the protective effects, UCN treatment of both neonatal and adult rat cardiomyocytes stimulates the secretion of atrial natriuretic peptide (ANP) and brain natriuretic peptide (BNP), both of which are markers of hypertrophy [90, 91]. Another significant contribution of UCN1-mediated cardioprotection involves de novo protein synthesis. For example, expression of the cardioprotective heat shock protein 90 (HSP90) has been reported to be induced by UCN1, a specific effect blocked by the MK1/2 inhibitor PD98059 [92]. The expression of cardiotrophin-1 (CT-1), an additional cardioprotective peptide, is also increased upon exposure of cells to UCN 1 [93].
Based on the above experimental evidence for the cardioprotective role of urocortins and on the reported role of CRH on various peripheral sites, a possible role for CRH in the cardiac adaptive response to stressors has recently been explored by our group. The absence of CRH in mice leads to low levels of ACTH and corticosterone and a blunted HPA response. Thus, CRH regulates adrenal function as well as responses to stress mediated via the HPA axis. Genetic ablation of CRH (Crh-knockout, Crh−/−, mice) leads to inability of the cardiovascular system to cope with a mild stressor, resulting in death. Similar to those described for UCNs, signaling pathways involved in this process, include Erk1/2, Akt, and AMPK. Crh deletion also results in increased infiltration of the heart tissue with inflammatory cells and apoptosis. Metabolic dysregulation evident by significantly compromised ability for fatty acid oxidation may underlie, at least, the latter, and possibly drive the compromised function of the Crh−/− heart [94].
14.4 Conclusion
Heart is a tissue where different pathways activated by various stressors convey and alter its functions, often resulting in remodeling, chronic inflammation, metabolic dysfunction and ultimately, heart failure. Given the active participation of the heart in the adaptive response to stressors, and the effects of specific mediators of the adaptive response on these processes, the possibility they represent promising targets for novel therapeutic schemes is raised. New models taking into account the contribution of these factors at both the cell and systemic responses and proposing novel therapeutic strategies need to be validated.
References
1.
4.
5.
6.
Davis AM, Natelson BH. Brain-heart interactions. The neurocardiology of arrhythmia and sudden cardiac death. Tex Heart Inst J. 1993;20:158–69.PubMedCentralPubMed
7.
8.
9.
10.
De Kloet ER, Vreugdenhil E, Oitzl MS, Joels M. Brain corticosteroid receptor balance in health and disease. Endocr Rev. 1998;19:269–301.PubMed
11.
12.
Mastorakas G, Chrousos GP, Weber JS. Recombinant interleukin-6 activates the hypothalamic-pituitary-adrenal axis in human. J Clin Endocrinol Metab. 1993;77:1690–4.
13.
14.
15.
16.
17.
18.
Rosmond R, Dallman MF, Björntorp P. Stress-related cortisol secretion in men: relationships with abdominal obesity and endocrine metabolic and hemodynamic abnormalities. J Clin Endocrinol Metab. 1998;83:1853–9.PubMed
19.
Grippo AJ, Johnson AK. Stress, depression and cardiovascular dysregulation: a review of neurobiological mechanisms and the integration of research from preclinical disease models. Stress. 2009;12:1–21.PubMedCentralPubMedCrossRef
20.
21.
22.
Engel FB, Schebesta M, Duong MT, Lu G, Ren S, Madwed JB, et al. p38 MAP kinase inhibition enables proliferation of adult mammalian Cardiomyocytes. Genes Dev. 2005;19:1175–87.PubMedCentralPubMedCrossRef
< div class='tao-gold-member'>
Only gold members can continue reading. Log In or Register a > to continue
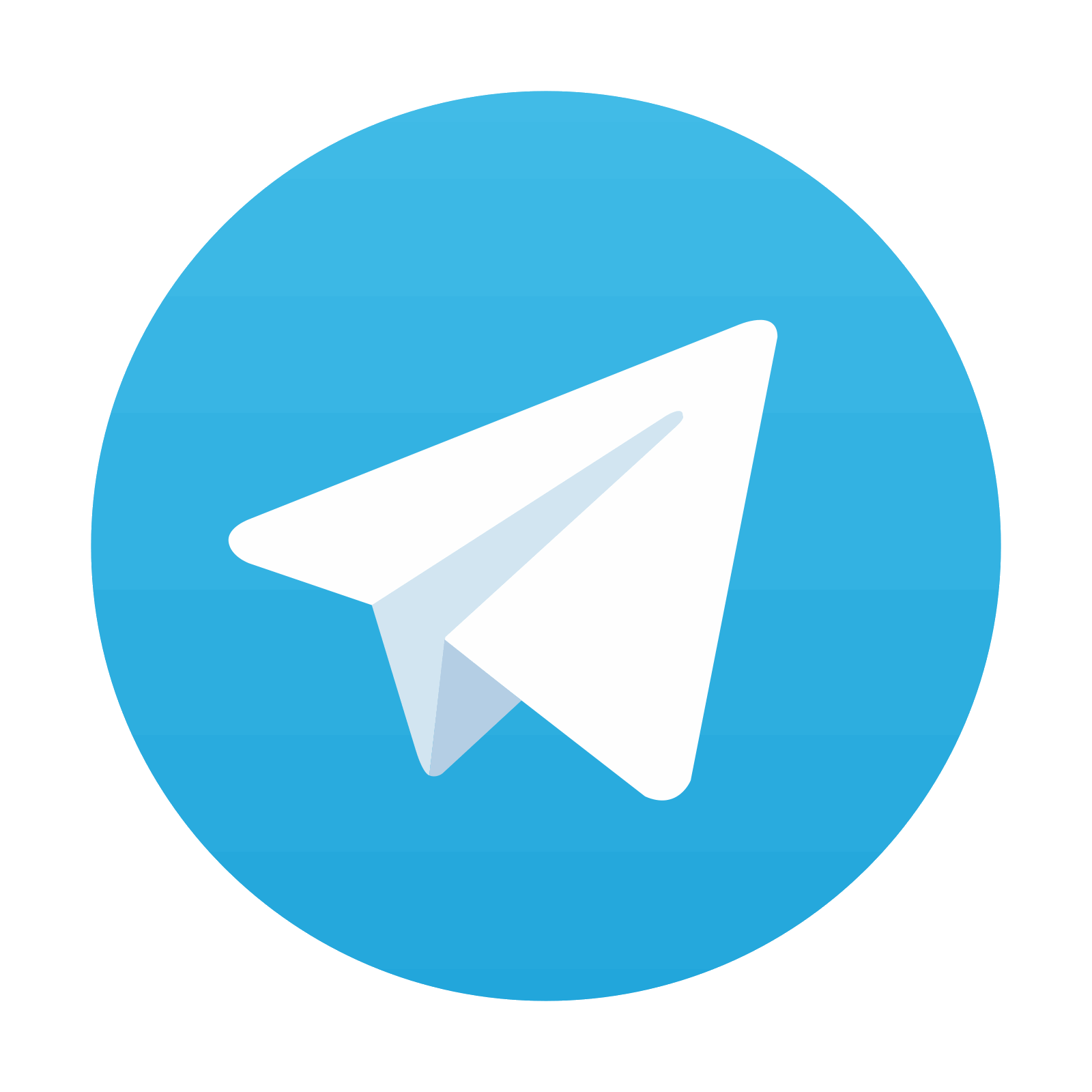
Stay updated, free articles. Join our Telegram channel
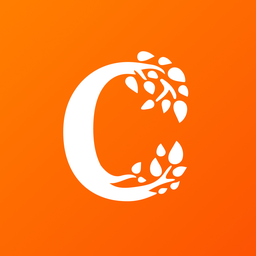
Full access? Get Clinical Tree
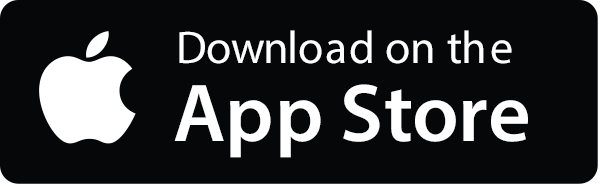
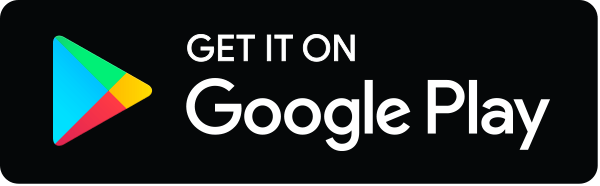