Strategies for Gene and Cell Therapy in Heart Failure
Shi Yin Foo
Anthony Rosenzweig
The advances in cardiovascular care in the last three decades have paradoxically led to an increase in the number of individuals with heart disease, as survival rates from previously fatal myocardial infarctions (MIs) have improved. Consequently, more patients suffer from repeated MIs, congestive heart failure, and clinically significant arrhythmias. Despite progress in pharmacologic and device-based therapies, heart failure remains a growing cause of morbidity and mortality. Most current therapies are aimed at mitigating disease progression rather than reversing the underlying causes. Thus, investigation of novel biological therapies for heart failure is driven by both a clinical need for more effective treatment and a desire to correct fundamental abnormalities in an attempt to reverse the pathology of heart failure.
Genetic therapies provide an opportunity for highly specific manipulation of molecular pathways for which no pharmacologic reagent may exist. Moreover, the ability to manipulate these pathways locally in the myocardium can obviate concerns about systemic side effects inherent in pharmacologic approaches (1). Cell replacement strategies offer an opportunity to restore the number and function of endogenous cell populations, including cardiomyocytes and endothelial cells. However, each of these strategies introduces new concerns not seen with traditional approaches including potentially fatal vector toxicities (2,3) or cell-induced ventricular arrhythmias (4).
Despite these concerns, there is cause for cautious optimism and careful consideration of these approaches is warranted. In this chapter, we describe progress in these growing fields as it relates to heart failure. We consider, in turn, gene- and cell-based strategies under active investigation.
Gene-Based Therapy: Overview
Since heart failure encompasses a wide variety of primary causes, from coronary insufficiency to idiopathic cardiomyopathies, gene therapy strategies may need to be tailored to the specific form of heart failure—one size, or gene, may not fit all. For example, strategies to induce the growth of new blood vessels in specific regions of the heart may make sense for ischemic but not nonischemic cardiomyopathies where effective therapy may require more global, myocyte-targeted therapy. However, common features seen in heart failure of many etiologies, such as myocyte loss and abnormalities of excitation-contraction coupling, are potentially attractive candidates for intervention that might be applicable to a variety of primary disorders. In any context, successful gene therapy will require three critical components appropriate to the disease target at hand: a vector for delivery of the therapeutic genetic material, an appropriate delivery system, and, perhaps most important, a validated molecular target that can be appropriately modulated.
Vectors
There have been significant improvements in the vectors available for gene transfer (5,6,7) and a growing number have been used in both experimental and clinical gene transfer experiments (Table 47-1). Importantly, some of the most commonly used systems are not applicable to cardiac gene transfer, which requires in vivo gene delivery (as compared to ex vivo gene transfer) to cells that are not generally replicating. Here we focus on vectors most relevant to cardiac gene transfer.
Table 47-1 Vectors for Gene Delivery | ||||||||||||||||||||||||||||||||||||
---|---|---|---|---|---|---|---|---|---|---|---|---|---|---|---|---|---|---|---|---|---|---|---|---|---|---|---|---|---|---|---|---|---|---|---|---|
|
Plasmid DNA
Multiple investigators have demonstrated that the heart can internalize and express genes injected directly as plasmid DNA (also termed naked DNA) to indicate the absence of a more elaborate packaging system. Use of plasmids avoids many biosafety concerns associated with viral vectors. However, both the level of transgene expression and the efficiency of gene transfer (percentage of target cells actually expressing the transgene) are generally much lower with unmodified plasmid DNA than with viral vectors or more elaborate chemical packaging systems. Nevertheless, muscle injection of plasmid vectors encoding secreted angiogenic factors has demonstrated significant biological effects in animals models (8,9,10).
A variety of modifications have been explored in the hope of enhancing the effectiveness of plasmid transfection without invoking the biosafety issues that accompany viral vectors. Liposomal preparations encase a DNA vector core with an artificial lipid bilayer that facilitates fusion with cell membranes (11). In some models, liposomal gene transfer to the heart appears effective despite a relatively low efficiency, perhaps because it evokes less local or inflammatory reaction than do adenoviral vectors (12). Other investigators have used a hybrid liposomal micelle containing envelope components of the HVJ virus (hemagglutinating virus of Japan or Sendai virus) to transfect a variety of cell types, including cardiomyocytes (13). However, transgene expression remains transient.
In another approach, liposomal-DNA micelles are attached to the phospholipid shell of gas-filled microbubbles, similar to those used as echocardiography contrast material. The mixture is injected intravenously and ultrasonography is used to cavitate the microbubbles while in the myocardial circulation. Cavitation causes a local shockwave that appears to enhance cell uptake of the plasmid (14). Newer strategies untested in the heart include peptide-cDNA heteroduplexes, in which the peptide contains a nuclear localization signal to enhance importation of the cDNA (15) and RNA-based peptide aptamers, which target and inhibit specific proteins (16). Finally, proteins that enhance cellular entry of exogenous material (such as the HIV tat protein) have been used to enhance both liposomal (12) and viral gene transfer (17).
Adenoviruses
Strategic advantages of recombinant adenoviral vectors for cardiac gene transfer include ease of preparation at extremely high titer and effective transduction of nonreplicating cells, including cardiomyocytes in vivo after direct injection or perfusion approaches (18). However, transient expression of introduced genes and induction of a potent host immune and inflammatory response limit their usefulness. Notably, up to 97% of individuals have pre-existing antibodies to common adenoviral serotypes (19) that can antagonize receptor binding, activate complement, and induce an inflammatory response (20). In addition, adenoviral infection of endothelial cells can upregulate adhesion molecules such as ICAM-1 and VCAM-1, leading to increased local infiltration of leukocytes (21) and the development of neointimal hyperplasia (22). These immune responses are likely responsible for decreasing the efficiency of the initial infection.
Another concern is the virtually unavoidable low-level contamination of recombinant adenoviral preparations with replication-competent adenovirus, likely generated through recombination events (23). This may be of particular concern for cardiac gene transfer since adenovirus has been isolated from cases of myocarditis and idiopathic dilated cardiomyopathy (IDCM) (24). Intriguingly, type 2 and 5 adenoviruses share a common receptor with the cox-sackie B viruses (25), a known cause of myocarditis. However, the pathogenic role of adenovirus in myocarditis is unproven and, to date, no cases of myocarditis have been reported after adenoviral cardiac gene transfer. New packaging cell lines that further separate genomic replication components may decrease the likelihood of wild-type virus generation by several orders of magnitude (26).
Cardiac gene transfer with original (first-generation) adenoviral vectors in animal models usually effects high-level transgene expression for approximately 1 week in vivo (18,27,28,29), at which point it is eliminated or markedly diminished by the host cellular immune response. A variety of approaches have been explored to mitigate these responses, including the use of agents such as cyclosporin A (30,31) or soluble CTLA-Ig (32), which inhibits antigen presentation to T cells. However, the potential side effects
of long-term immunosuppression dampen enthusiasm for this as a clinical solution. Since some of the host response is directed to viral antigens, considerable effort has been directed at attenuation of adenoviral gene expression by introduction of mutations in additional early adenoviral genes [so-called second-generation vectors (33)] or engineered deletion of virtually all viral genes, as in so-called gutless vectors (34). Both of these approaches prolong transgene expression and further decrease the likelihood of recombination-mediated generation of replication competent adenovirus. Interestingly, in some instances retaining specific adenoviral genes can actually reduce the host response (e.g., retention of the E3 region mitigated inflammation and neointima formation after vascular gene transfer in vivo) (35). Currently, little is known about the long-term or clinical effects of second-generation and gutless adenoviral vectors in the cardiovascular system.
of long-term immunosuppression dampen enthusiasm for this as a clinical solution. Since some of the host response is directed to viral antigens, considerable effort has been directed at attenuation of adenoviral gene expression by introduction of mutations in additional early adenoviral genes [so-called second-generation vectors (33)] or engineered deletion of virtually all viral genes, as in so-called gutless vectors (34). Both of these approaches prolong transgene expression and further decrease the likelihood of recombination-mediated generation of replication competent adenovirus. Interestingly, in some instances retaining specific adenoviral genes can actually reduce the host response (e.g., retention of the E3 region mitigated inflammation and neointima formation after vascular gene transfer in vivo) (35). Currently, little is known about the long-term or clinical effects of second-generation and gutless adenoviral vectors in the cardiovascular system.
Adeno-Associated Viruses
Recombinant adeno-associated viruses (rAAV) appear particularly promising for cardiac gene transfer (5,36,37) for several reasons. They are derived from parvoviruses, which are not known to be pathogenic in humans and generally do not evoke a vigorous cellular immune response. rAAV achieve transgene expression lasting months in many systems (36,38,39). Cardiac injection of rAAV generally produces less initial but more sustained transgene expression in comparison to the adenoviral vectors previously discussed (5,36,37). Thus, rAAV may be the vector of choice for sustained expression of secreted gene products. However, less is known regarding the ability of rAAV to mediate sufficient transgene expression to modulate overall heart function, as reported with adenoviral vectors (40). As with newer-generation adenoviral vectors, the production and purification of sufficient clinical grade rAAV remain challenging.
A growing number of adeno-associated viruses (AAV) serotypes have been isolated (41,42). AAV2 has been the most commonly used AAV vector, although its efficiency for cardiomyocytes appears relatively low, with ∼25% of the cardiomyocytes within the area of cardiac injection expressing the transgene at 1 month (43). The efficiency of AAV2 gene transfer can be limited by pre-existing antibodies that result from prior wild-type infection (19). The prevalence of anti-AAV2 antibodies in human serum can be as high as 35% to 80%, depending on the population (44). The adaptive, cell-mediated immune response is also activated by AAV but is much weaker than with adenovirus. This, in combination with the ability of AAV genomes to integrate, likely accounts for the dramatically improved duration of transgene expression after AAV gene transfer.
To enhance tissue specificity, recombinant AAV serotypes have been generated that use the AAV2 genome packaged into the viral capsids of other serotypes (43,45). Of these, recombinant AAV1 vectors carrying the AAV2 internal terminal repeats (ITR) sequences [rAAV1 (2) vectors] transduced adult cardiomyocytes both in vitro and in vivo at the highest frequency. In vivo, the efficiency was approximately 40% of cardiomyocytes within a 5-mm radius of the injection site 1 month after injection, but less than 10% at 1 year after intervention (43). This approach may be ideally suited to gene products that initiate a cascade of effects and can act in a paracrine manner. Another advantage of the rAAV1 (2) vectors is the relative lack of exposure of humans to the AAV1. In general, human serum lacks neutralizing antibodies to the AAV1 virus. In one study, only 20% of healthy individuals harbored anti-AAV1 antibodies and these antibodies were less potent than anti-AAV2 antibodies in preventing viral infection (46). However, development of a humoral immune response would likely hamper attempts at repeated gene replacement using the same vector.
AAV8 appears to have a robust tropism for striated muscle, including the heart (47). Interestingly, this particular serotype outperforms AAV1 in cardiac muscle transduction after intravenous administration, but not after intramuscular injection in mice, suggesting that an additional barrier to successful gene transfer in vivo is the ability to traverse the blood vessel wall (47).
AAV vectors, in general, mediate a relatively low-level transgene expression that can require weeks to reach its peak. Recently, Samulski et al. have developed AAV vectors encoding a single-stranded, self-complementary DNA strand that substantially accelerates and enhances transgene expression (48). However, the use of AAV is also limited by the small size of the virus, which severely constrains the amount of DNA that can be packaged (<5 kb). Self-complementing AAV, which essentially encode both strands in one molecule, reduce this amount by approximately twofold.
Lentiviruses
Although derived from a family of retroviruses that includes human and feline immunodeficiency viruses, lentiviruses can infect both dividing and nondividing cells, in contrast to other retroviruses which infect only actively replicating cells. This distinction is critical to their ability to transduce cardiomyocytes. Lentiviruses encode an RNA genome, which is converted to DNA and integrated into the host chromosome. Lentiviruses have specific tropisms that restrict their host cell range, but this can be overcome by pseudotyping the viral envelope through incorporation of the envelope from other retroviruses (particularly vesicular stomatitis virus, which has a broad host range). Integration into the host genome enhances the stability of transgene expression but also carries the risk of potentially serious adverse effects. These effects can arise either through insertional mutagenesis or transactivation of neighboring genes, as seen in recent clinical trials of retroviral gene therapy (2,49). Lentiviral gene vectors have been successfully employed in a wide variety of cell types, including cardiomyocytes (50,51,52,53,54,55).
Advanced-generation lentiviruses achieve gene transfer efficiencies in cardiomyocytes comparable to that of adenoviral vectors. These lentiviruses contain elements of the HIV pol gene as a nuclear transport signal and an mRNA-stabilizer from the woodchuck hepatitis virus to increase integration (56). Transduction efficiency in cultured rat cardiomyocytes was over 80%. After delivery of these lentiviral vectors through coronary perfusion to the rat heart after aortic clamping, 85% of cardiomyocytes expressed the reporter transgene at 15 days (56).
Lentiviruses elicit relatively little immune response to intrinsic viral proteins, although the use of lentivirus in vivo
may be limited by complement activation acutely (57). The adaptive, cytotoxic immune response that limits long-term expression appears primarily directed against the exogenous transgenes. Complement activation can be mitigated somewhat by using different pseudotypes; vesicular stomatits virus capsid proteins appear particularly prone to complement activation. In some studies, pseudotyping with more exotic envelope proteins, such as those from Ebola and Mokola viruses, allows for higher infection efficiency in cardiomyocytes in vivo (54). However, the immune response to these recombinant vectors has not been fully characterized.
may be limited by complement activation acutely (57). The adaptive, cytotoxic immune response that limits long-term expression appears primarily directed against the exogenous transgenes. Complement activation can be mitigated somewhat by using different pseudotypes; vesicular stomatits virus capsid proteins appear particularly prone to complement activation. In some studies, pseudotyping with more exotic envelope proteins, such as those from Ebola and Mokola viruses, allows for higher infection efficiency in cardiomyocytes in vivo (54). However, the immune response to these recombinant vectors has not been fully characterized.
Herpes Virus/Amplicons
Herpes simplex 1 (HSV-1) can infect both dividing and nondividing cells and has a broad host cell range although it has a strong tropism toward neurons. The wild-type virus has a large genome and, thus, vectors derived from HSV-1 can accommodate large transgenes (<35 kb). This feature has been exploited for cardiac gene transfer for large genes such as the ryanodine receptor and titin (58), which would not be feasible with most other vector systems. Two types of HSV-1-based vectors are currently in use. These include vectors produced by inserting exogenous genes into the viral backbone, and HSV amplicon virions that are produced by inserting transgenes into a plasmid carrying the HSV origin of replication and packaging signal.
Toxicity
The previous discussion above and Table 47-1 should make clear that no vector is ideal; each brings limitations and potential toxicities. These toxicities have led to a concerning number of complications in early clinical experience. One of the most widely publicized involved a 17-year-old patient with partial ornithine transcarbamylase (OTC) deficiency, an X-linked defect of the urea cycle which causes a variety of neurologic symptoms including seizures and mental retardation (59). Therapy for the condition relies on alternative substrate administration but mortality rates with the disease are high. Following the administration of an adenoviral vector encoding carrying OTC, this patient developed acute respiratory distress syndrome, ultimately dying of multiple organ failure. This patient developed a systemic inflammatory syndrome with activation of the complement cascade that contributed to his demise (60,61). Thus, the host immune response mounted to viral vectors can have catastrophic consequences. In another instance, ex vivo retroviral gene transfer to bone marrow stem cells led to long-term remission in a small number of children (2) but was complicated by clonal, leukemic T-cell proliferation apparently related to construct insertion inducing aberrant proto-oncogene expression (2). While such insertional effects had always been considered a theoretical possibility with integrating vectors, the surprisingly high frequency of the event [occurring in two of the first 10 treated patients (2)] is obviously of great concern. Approaches to minimizing this risk currently being investigated include targeting viral integration to specific sites in the genome (62), use of chromatin-insulator elements to prevent the nonspecific activation of nearby oncogenes (63), and inclusion of inducible suicide genes to permit targeted destruction of transformed cells (64).
Delivery Methods
Many of the issues concerning delivery are common to both genetic and cell-based therapies and will be addressed together in this section. A variety of systems have been explored in animal models (53,65,66,67,68,69), while clinical experience is more limited. Some techniques successfully employed in animals may not be applicable to the sick cardiac patients most in need of such therapies. As with the choice of vector, the optimal delivery method also depends on disease context and the therapeutic target. The focal transgene expression achieved with direct injection (whether surgical or catheter-based) may be appropriate for angiogenic therapy to hypoperfused myocardial segments in ischemic cardiomyopathy, while delivery of cell-autonomous transgenes to improve cardiomyocyte function in nonischemic cardiomyopathy likely requires more diffuse delivery.
Systemic
In the simplest approach, genes or cells are injected intravenously. However, this approach often suffers from the twin evils of inadequate delivery to the target organ and extraneous, possibly detrimental delivery to unintended tissues. For gene delivery, systemic intravenous delivery usually results in either extremely low or undetectable levels of cardiac transgene expression. In contrast, certain cell populations (such as bone marrow-derived angioblasts) have been shown to home preferentially to areas of ischemic injury (70). Nevertheless, systemic delivery of cells will likely result in delivery of fewer cells to the heart than will direct delivery. Ex vivo expansion of the cell populations could be used to overcome this barrier but may heighten concerns over inappropriate homing to other sites.
Intramyocardial Direct Injection
Direct injection of vectors or cells into the myocardium introduces high concentrations of the therapeutic agent in a highly localized manner. The exposure of the region at risk to these agents is not limited by cell or vector uptake through the vascular system but does, in general, entail more invasive procedures such as transendocardial or transcoronary vein injection during catheterization, or epicardial injection during cardiac surgery (4,71).
Direct injection has several advantages. First, injection can be limited to regions mostly likely to benefit while minimizing adverse effects in other regions or systemically. Second, in patients with coronary stenoses, direct injection provides access to hypoperfused myocardium excluded from artery-based perfusion approaches. Third, intramyocardial delivery obviates concerns in cell-based therapy that coronary infusion could pose an embolic risk in the small-caliber vessels of the heart. These features likely account for the fact that direct injection was one of the first techniques utilized clinically for both genes (72) and cells (73).
The highly focal nature of delivery may necessitate multiple injections to cover the entire region at risk, increasing the risk of mechanical injury or perforation. Direct injection of cells may lead to islands of cells that are isolated from an adequate blood supply and are thus unlikely to survive. In addition, patients most in need of such rescue therapies may be the individuals least likely to do well due to the invasiveness of these procedures. Finally, this approach will generally not be appropriate for conditions and molecular targets that require delivery of therapy to a majority of the heart.
Perfusion-Based approaches
A variety of catheter designs permit cannulation and perfusion of genes or cells down a coronary artery with or without occlusive balloon inflation. Some investigators have reported that myocardial gene delivery with this approach is quite efficient (74,75,76), although systemic vector delivery can also be substantial. A variation of coronary-mediated delivery is whole-heart perfusion with cross-clamping of the aorta alone or in combination with the pulmonary artery, first performed successfully in rodents (66,77). This approach achieves diffuse, relatively homogeneous myocardial transduction and is capable of modulating the overall intrinsic contractile properties of the heart (66,78). Extrapolation of these studies to human disease could entail perfusion in association with cardiopulmonary bypass (79) or retroperfusion via catheters (80).
Targets for Gene Therapy in Heart Failure
While some approaches to therapeutic gene delivery focus on the underlying cause of heart failure (as in the case of angiogenesis), the diverse etiologies that can contribute to heart failure, as well as the usual long delay between these inciting events and clinical presentation, represent significant challenges to this approach. For these reasons, many strategies have targeted features common to multiple forms of heart failure, including loss of cardiomyocytes through programmed cell death, abnormalities of excitation-contraction coupling and calcium handling, as well as dysfunctional adrenergic signaling (Table 47-2).
Improving Cardiac Perfusion
The most common contributor to heart failure in the United States is ischemic injury related to coronary artery disease (CAD). Therapeutic angiogenesis is directed at improving myocardial perfusion, particularly in patients for whom traditional percutaneous or surgical options have been exhausted. Currently, these efforts have been directed at relieving symptoms of ischemia, although the potential to improve cardiac function has been suggested by animal models, underscoring the close connection between angiogenic pathways and cardiac function (81). In animals, vascular endothelial growth factor (VEGF), fibroblast growth factor-2 (FGF-2), hepatocyte growth factor, or hypoxia-inducible factor-1 (HIF-1) (82) can induce neovascularization. In some animal models, new vessel formation is accompanied by improvements in myocardial contractile function (83,74). Early uncontrolled trials have suggested a reasonable safety profile (84). Although small numbers of patients have improved after direct myocardial delivery of VEGF (72,84), efficacy cannot be inferred in the absence of contemporaneous controls. It seems likely that extrapolation to the heart failure population awaits evidence from large, randomized, placebocontrolled trials.
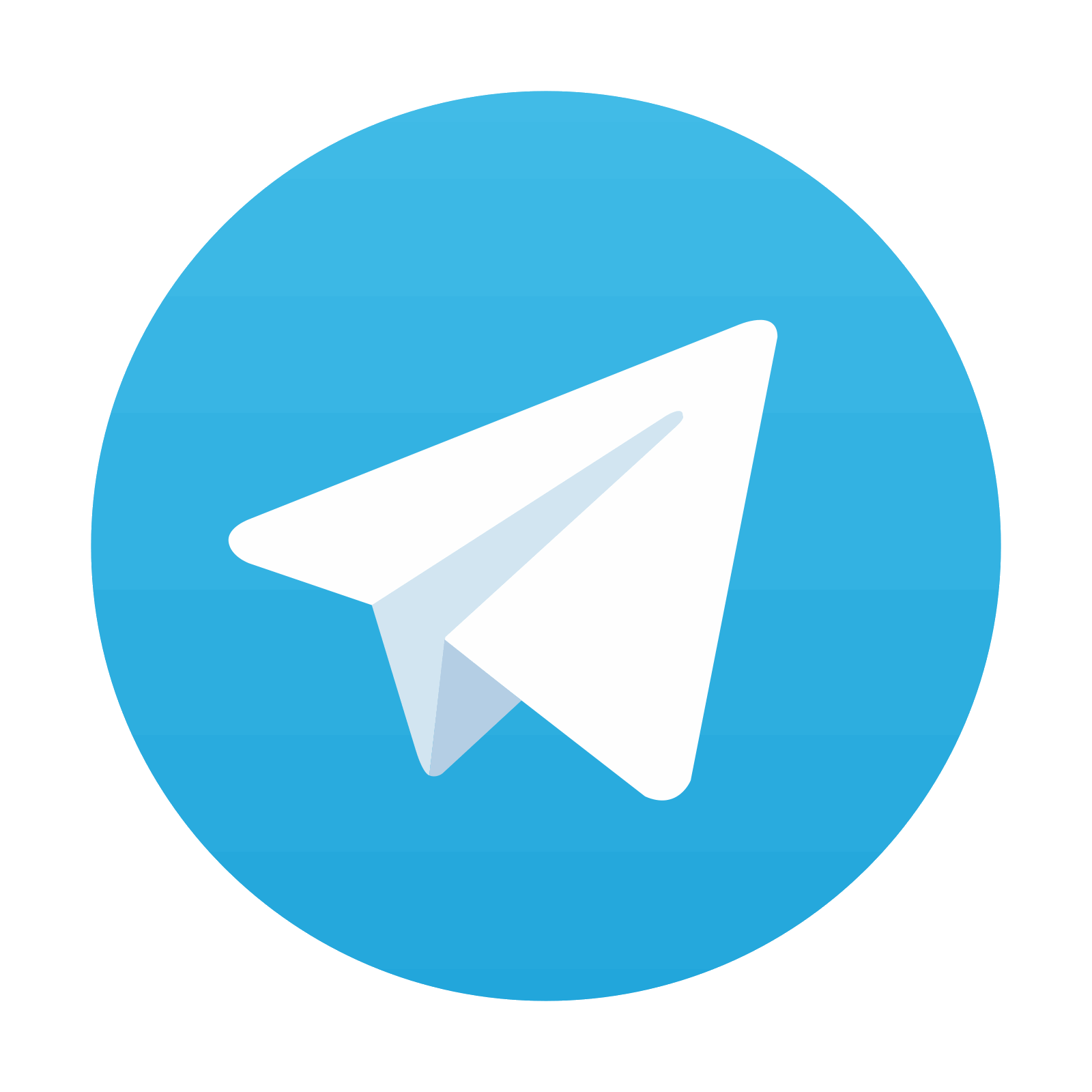
Stay updated, free articles. Join our Telegram channel
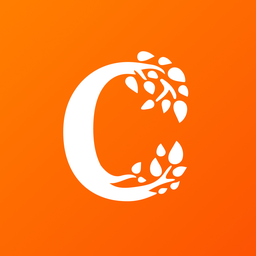
Full access? Get Clinical Tree
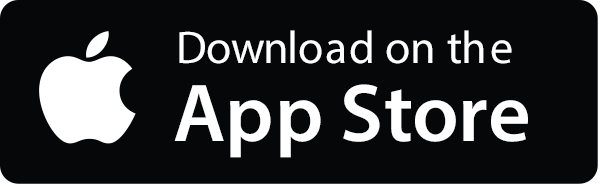
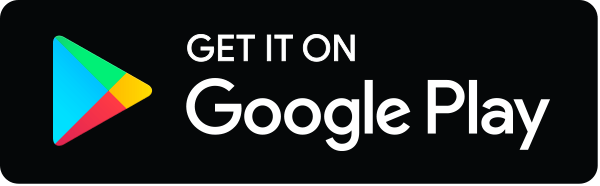