Fig. 8.1
Paracrine effect of stem cells thought to improve cardiac function after injury
8.5.1 Angiogenesis and Revascularization
Angiogenesis is widely regarded as an attractive approach to treat ischemic heart disease, for example to meet demands for blood supply during pathological hypertrophy, peripheral artery disease, and after MI (Khurana et al. 2005; Oka et al. 2014). In recent years it has been demonstrated that pro-angiogenic factors are readily secreted by stem cells. For MSCs it has been demonstrated that it contains vascular endothelial growth factor (VEGF ) , fibroblast growth factor 2 (FGF-2), interleukin-6 (IL-6), placental growth factor, angiopoietin-1 (Ang-1), monocyte chemoattractant protein-1, placental growth factor (PLGF) , hepatocyte growth factor (HGF) , transforming growth factor β (TGF-β), MYDGF, and cysteine-rich angiogenic inducer 61 (Cyr61) (Boomsma and Geenen 2012; Chen et al. 2008; Estrada et al. 2009; Hung et al. 2007; Kinnaird et al. 2004; Korf-Klingebiel et al. 2015; Rehman et al. 2004; Wu et al. 2007). The secretion of angiogenic factors by MSCs can be upregulated by a range of stimuli such as transforming growth factor α (TGF-α) (De Luca et al. 2011), tumor necrosis factor α (TNF-α) , LPS, hypoxia (Crisostomo et al. 2008; Rehman et al. 2004), epidermal growth factor (EGF) (Kilroy et al. 2007), and serum starvation (Oskowitz et al. 2011). Besides growth factors and cytokines it has been suggested stem cells release exosomes that can promote angiogenesis via microRNAs such as miR-126, miR-130a, miR-132, and miR-146 (Ailawadi et al. 2015; Cervio et al. 2015; Das and Halushka 2015; Emanueli et al. 2015). The pro-angiogenetic effect of the stem cell secretome or individual factors can be tested in assays such as endothelial cell proliferation and migration assays, HUVEC-tube-formation assay, chicken chorioallantoic membrane (CAM) assay , and the mouse matrigel plug assay (Bronckaers et al. 2014). That MSCs and their secretome promote angiogenesis in vivo after cardiac injury has been demonstrated in a large number of independent studies (e.g. Nagaya et al. 2004; Nagaya et al. 2005; Takahashi et al. 2006; Timmers et al. 2011).
8.5.2 Anti-apoptotic Effects
Ischemic diseases induce apoptosis of functional cells, such as cardiomyocytes, due to the lack of oxygen and nutrients. Thus, anti-apoptotic therapies can delay cell death providing more time for angiogenesis to supply the injured tissue. A large number of studies has shown that anti-apoptotic therapies significantly protect the heart from ischemic injury (Lavu et al. 2011). That stem cells secret in addition to pro-angiogenic also anti-apoptotic factors has been shown for MSCs by Gnecchi and coworkers (Gnecchi et al. 2005, 2009) and for adipose-derived stem cells by Rehman and coworkers (Rehman et al. 2004). Key pro-survival factors secreted by MSCs are for example the secreted frizzled related protein 2 (Sfrp2) (Mirotsou et al. 2007), insulin growth factor 1 (IGF-1), and platelet-derived growth factor PDGF (Takahashi et al. 2006). As for angiogenic factors, the cardioprotective effect of the stem cell secretome could be enhanced by a range of stimuli such with TGF-β or by activating the tumor necrosis factor receptor-2 (Herrmann et al. 2010). Finally, it has been shown that exosomes from human cardiac progenitor cells inhibit cardiomyocyte apoptosis and improve cardiac function after MI via miR-210 (Barile et al. 2014).
8.5.3 Anti-remodeling
Inflammatory cells infiltrate the heart after an MI, interstitial cells proliferate, and a subset of fibroblasts differentiates into myofibroblasts depositing extracellular matrix (ECM) resulting in the replacement of the necrotic myocardium with scar tissue. The initial inflammatory phase of scarring helps to maintain the tensile strength and integrity of the heart. Consequently, inhibition of this process leads to increased incidence of cardiac rupture or ventricular dilatation (Seropian et al. 2014). However, it has to be noted that ECM remodeling is a highly delicate and well-orchestrated process. For example, it is initially important to degrade the existing ECM by activation of latent matrix metalloproteinases (MMPs) to promote the infiltration of inflammatory cells. During scar maturation, fibroblasts and vascular cells in the scar undergo apoptosis and a collagen-rich scar is formed that promotes both infarct expansion and extension leading to overall remodeling and change in the shape of the ventricle (Leask 2015; Sun et al. 2002). Successful wound healing and remodeling after a MI thus requires a delicate balance of collagen production as well as degradation.
Even though many stem cell-based studies report reduced fibrosis and improved remodeling, it is poorly understood which factors are responsible for this improvement and what the underlying mechanism is. One major focus in modulating (e.g. after MI) or preventing (e.g. hypertension and diabetes mellitus) cardiac fibrosis is to understand the underlying mechanisms of myofibroblast differentiation. It is known that this process is highly regulated by extracellular factors such as TGF-β, endothelin-1 (ET-1), angiotensin II (Ang II), connective tissue growth factor (CCN2), and PDGF (Leask 2015). Thus, it is likely that the secretome of stem cells has also an influence on cardiac remodeling by acting on myofibroblast differentiation and thus the regulation of collagen biosynthesis and maturation. Mias and co-workers have shown that conditioned medium of MSCs reduce the proliferation of fibroblasts, represses alpha-smooth muscle actin expression and collagen secretion, and promotes matrix metalloproteinase secretion. The work of He and coworkers has shown that Sfrp2, known to be secreted by MSCs (Mirotsou et al. 2007), reduces fibrosis and improves cardiac function via its inhibition of bone morphogenetic protein 1 (Bmp1)/tolloid-like metalloproteinase, a key regulator of collagen biosynthesis and maturation after tissue injury (He et al. 2010).
8.5.4 Metabolism
The heart requires ATP for contraction and has a very high-energy demand. During heart development cardiac metabolism switches from predominantly glycolysis and glucose oxidation in fetal cardiomyocytes to β-oxidation of fatty acids in adult cardiomyocytes (Gnecchi et al. 2008; Stanley et al. 2005). Yet, after MI the heart undergoes again metabolic alterations switching back to increased glucose uptake. This results in a high-energy phosphate content and phosphocreatine-to-ATP ratio in the infarct border zone, which is believed to correspond to the severity of left ventricular contractile dysfunction and left ventricular remodeling (Hu et al. 2006; Stanley et al. 2005). Gnecchi and coworkers have shown that MSCs overexpressing Akt, but not unmodified MSCs, can prevent cardiac metabolic remodeling following MI (Gnecchi et al. 2009). Subsequently, Hughey and coworkers have reported that also transplantation of non-modified MSCs enhanced the energetic state in the infarcted heart. Utilizing hyperinsulinemic-euglycemic clamps, coupled with 2-[(14)C]deoxyglucose administration, they demonstrated that the “improved systolic performance in MSC-treated mice was associated with a preservation of in vivo insulin- stimulated cardiac glucose uptake” and insulin signaling (Hughey et al. 2014).
8.5.5 Immune and Inflammatory Modulation
Efforts to elucidate the underlying mechanisms of tissue regeneration revealed the importance of immune cell types in promoting a permissive local environment for effective cell replacement and restoration of tissue integrity (Forbes and Rosenthal 2014). In addition, it has been shown that modulation of the immune system can prevent cardiac remodeling. For example, IL-1 or MCP-1 knockout mice exhibit markedly reduced dilative remodeling after MI, without affecting infarct size (Bujak et al. 2008; Dewald et al. 2005). In contrast, interleukin-1 receptor-associated kinase (IRAK)-M knockout mice had enhanced adverse remodeling and worse systolic dysfunction indicating that “IRAK-M attenuates adverse postinfarction remodeling suppressing leukocyte inflammatory activity, while inhibiting fibroblast-mediated matrix degradation” (Chen et al. 2012).
Xenotransplantation experiments suggested in 2000 that MSCs possess the ability to modulate the immune system (Liechty et al. 2000). Subsequently, Di Nicola and coworkers showed that MSCs can suppress the cell-mediated immune responses by inhibiting proliferation of CD4+ and CD8+ T cells (Di Nicola et al. 2002). Meanwhile it is clear that MSCs modulate different stages of the immune system namely antigen recognition and presentation, T cell activation, proliferation, and differentiation, and the T-cell effector stage (Aurora and Olson 2014; Kim and Cho 2015; Liang et al. 2014). For example, by releasing cytokines such as HLA-G5, prostaglandin E2 (PGE2), and indoleamine 2,3-dioxygenase (IDO) MSCs modulate the differentiation of monocytes. Secretion of HGF, IDO, PGE2, inducible nitric-oxide synthase, TGFβ1, and heme oxygenase-1 blocks T-cell activity (Mandi and Vecsei 2012; Uccelli et al. 2008; Zhao et al. 2008). In the beginning it was believed that MSCs are solely immunosuppressive (Fibbe et al. 2007; Selmani et al. 2008). For example, Lee and coworkers showed that intravenous human MSCs improve MI in mice because MSCs embolized in the lung are activated to secrete the powerful anti-inflammatory factor tumor necrosis factor-stimulated gene 6 protein (TSG-6) (Lee et al. 2009). Yet, it has become clear that they can also stimulate the release of pro-inflammatory molecules (Bernardo and Fibbe 2013; Frangogiannis 2012).
8.5.6 Activation of Endogenous Repair Processes
The heart has for a long time been considered as a post-mitotic organ, meaning that shortly after birth no new cardiomyocytes are formed, neither by cardiomyocyte proliferation nor by stem cell differentiation. However, recent data suggest that there is modest cardiomyocyte turnover (around 1 %) in adult mouse and human hearts (Bergmann et al. 2015; Senyo et al. 2013). The current hypothesis is that new cardiomyocytes are predominantly added by proliferation of pre-existing cardiomyocytes. Recently, fate mapping of hypoxic cells and their progenies identified a “rare population of hypoxic cardiomyocytes that display characteristics of proliferative neonatal cardiomyocytes, such as smaller size, mononucleation and lower oxidative DNA damage” (Kimura et al. 2015). These cells might present a subpopulation of cardiomyocytes utilized for cardiac homeostasis. Whether the secretome can promote endogenous stem cells to differentiate into cardiomyocytes or enhances proliferation of a subpopulation of cardiomyocytes remains unclear. However, it has for example been shown that factors such as FGF1 can promote cardiomyocyte proliferation (Engel et al. 2005) and improve cardiac function (Engel et al. 2006). Even though some studies indicate cell cycle activation of cardiomyocytes upon stem cell transplantation there is currently no evidence for the formation of new cardiomyocytes in stem cell-based therapies.
8.6 Cell-Free Therapy
Growth factors and cytokines have due to their pleiotropic effects long before stem-cell based approaches been considered for heart therapies. For example, the beta isoform of Neuregulin-1 (NRG-1β) exhibits anti-fibrotic effects in several animal models of heart failure (Galindo et al. 2014b) including diabetic cardiomyopathy in a rat model (Li et al. 2013) and MI in swine (Galindo et al. 2014a). NRG-1β has also been demonstrated in clinical trials to have beneficial effects by maintaining cardiac structure and function, as well as mediating reverse remodeling (Galindo et al. 2014b; Rupert and Coulombe 2015). NRG-1β exhibits its positive effects for example by enhancing cardiomyocyte cell survival (Fukazawa et al. 2003), angiogenesis (Russell et al. 1999), and excitation-coupling (Timolati et al. 2006). In addition, Galindo and coworkers have demonstrated that NRG-1β reduces the number of myofibroblasts (Galindo et al. 2014a; Pentassuglia and Sawyer 2013).
Several clinical trials have been initiated to investigate the potential of cytokines and growth factors such as VEGF-165, VEGF-121, VEGF-2 (VEGF-C), FGF-4, granulocyte colony stimulating factor (GCSF) , EPO, and IGF-1 for treating cardiovascular diseases focusing on angiogenesis (Beohar et al. 2010; Engelmann et al. 2006; Formiga et al. 2012; Hwang and Kloner 2010; Ripa et al. 2006). However, the results have been disappointing with no or minor effects, which has been attributed, at least partially, to the short-lived effect and high instability of proteins after intravenous infusion or intracoronary delivery (Formiga et al. 2012). A major problem in these therapies besides limited protein stability is the need of a distinct dose at a distinct location of a factor to exert a positive effect. For example, pro-angiogenic factors in to high doses can lead to the formation of aberrant and leaky vessels (Carmeliet 2005), hypotension (Eppler et al. 2002), and tumor angiogenesis (Epstein et al. 2001). In addition, the timing of therapy is an important factor. For example, IL6, a pleiotropic cytokine activating immune cells, acts after an MI protective and limits cardiac damage. Yet, if IL6 remains chronically activated it is pathogenic leading to chronic inflammation and fibrosis (Fontes et al. 2015). The importance of the positive early effect has been demonstrated by catastrophic effects upon broad inhibition of the inflammatory reaction in patients with MI using systemic corticosteroids (Roberts et al. 1976). Subsequent studies targeting specific immune pathways demonstrated that inhibition of adhesion molecules, chemokines and cytokines protects the ischemic and reperfused myocardium, reducing the size of the infarct by 40–50 %. Yet, clinical trials did not meet the expectations (Frangogiannis 2014). Another good example for the importance of time point of injection and duration of a treatment has been provided by Kang and coworkers describing the efficiency of GCSF therapy depending on timing and duration of the therapy (Kang et al. 2004).
Collectively, it is important to optimize the delivery strategy of secreted factors in regards to concentration and location. Recently first strategies have been developed. For example, gene constructs have been designed that allow cardiac-specific expression upon hypoxia (Su et al. 2004; Tang 2005). Such constructs can be delivered by adeno-associated viruses utilizing a catheter or as plasmids bound to microbubbles, which can be released by ultrasound-targeted microbubble destruction (Fujii et al. 2009; Suzuki et al. 2008). In addition, novel materials are continuously developed for controlled release of proteins and DNA (Jo and Tabata 2015; Yockman et al. 2008).
8.7 Outlook
Taken together, neither the delivery of single growth factors or cytokines nor the use of stem cells such as MSCs have been shown to have a significant beneficial effect on cardiac function. However, stem cells can supply secreted factors with the advantage of sustained pharmacokinetics, synergy from multiple factors, and opportunity for systemic infusion and thus repeated dosing. Thus, a valid approach to treat cardiovascular diseases might be the enhancement of stem cells by preconditioning or genetic manipulation to design cells that secrete multiple cytokines/growth factors at different concentrations to act synergistically at a distinct localization (MSCs will be homed to the correct place). These cells are called “Second-generation” stem cells (Nunez Garcia et al. 2015; Tran and Damaser 2015) and could be thought of as combinatorial drug manufacture and delivery mechanism. In addition, the analysis of stem cell secretomes holds promise to identify additional important factors for cardiac repair.
However, stem cell-based therapies have significant disadvantages related to immune compatibility, tumorigenicity, and the transmission of infections and they are time consuming (stem cell expansion and maintenance). Thus, it is recommended to continue to work on multi-factor therapies focusing on establishment of new technologies to overcome hurdles like protein stability, local application, release kinetics, and concentration. This would allow the preparation of large quantities of doses of cytokine/growth factor cocktails in an allogeneic or off-the-shelf fashion and thus treatment when needed including in acute conditions such as MI.
References
Ailawadi S, Wang X, Gu H, Fan GC (2015) Pathologic function and therapeutic potential of exosomes in cardiovascular disease. Biochim Biophys Acta 1852:1–11PubMed
Aurora AB, Olson EN (2014) Immune modulation of stem cells and regeneration. Cell Stem Cell 15:14–25PubMedCentralPubMed
Bannai H, Tamada Y, Maruyama O, Nakai K, Miyano S (2002) Extensive feature detection of N-terminal protein sorting signals. Bioinformatics 18:298–305PubMed
Barile L, Lionetti V, Cervio E, Matteucci M, Gherghiceanu M, Popescu LM, Torre T, Siclari F, Moccetti T, Vassalli G (2014) Extracellular vesicles from human cardiac progenitor cells inhibit cardiomyocyte apoptosis and improve cardiac function after myocardial infarction. Cardiovasc Res 103:530–541PubMed
Bartosh TJ, Ylostalo JH, Mohammadipoor A, Bazhanov N, Coble K, Claypool K, Lee RH, Choi H, Prockop DJ (2010) Aggregation of human mesenchymal stromal cells (MSCs) into 3D spheroids enhances their antiinflammatory properties. Proc Natl Acad Sci U S A 107:13724–13729PubMedCentralPubMed
Bendtsen JD, Jensen LJ, Blom N, Von Heijne G, Brunak S (2004a) Feature-based prediction of non-classical and leaderless protein secretion. Protein Eng Des Sel 17:349–356PubMed
Bendtsen JD, Nielsen H, von Heijne G, Brunak S (2004b) Improved prediction of signal peptides: SignalP 3.0. J Mol Biol 340:783–795PubMed
Beohar N, Rapp J, Pandya S, Losordo DW (2010) Rebuilding the damaged heart: the potential of cytokines and growth factors in the treatment of ischemic heart disease. J Am Coll Cardiol 56:1287–1297PubMedCentralPubMed
Bergmann O, Zdunek S, Felker A, Salehpour M, Alkass K, Bernard S, Sjostrom SL, Szewczykowska M, Jackowska T, Dos Remedios C, Malm T, Andrä M, Jashari R, Nyengaard JR, Possnert G, Jovinge S, Druid H, Frisén J (2015) Dynamics of cell generation and turnover in the human heart. Cell 161:1566–1575PubMed
Bernardo ME, Fibbe WE (2013) Mesenchymal stromal cells: sensors and switchers of inflammation. Cell Stem Cell 13:392–402PubMed
Bi B, Schmitt R, Israilova M, Nishio H, Cantley LG (2007) Stromal cells protect against acute tubular injury via an endocrine effect. J Am Soc Nephrol 18:2486–2496PubMed
Boden M, Hawkins J (2005) Prediction of subcellular localization using sequence-biased recurrent networks. Bioinformatics 21:2279–2286PubMed
Boomsma RA, Geenen DL (2012) Mesenchymal stem cells secrete multiple cytokines that promote angiogenesis and have contrasting effects on chemotaxis and apoptosis. PLoS One 7, e35685PubMedCentralPubMed
Bronckaers A, Hilkens P, Martens W, Gervois P, Ratajczak J, Struys T, Lambrichts I (2014) Mesenchymal stem/stromal cells as a pharmacological and therapeutic approach to accelerate angiogenesis. Pharmacol Ther 143:181–196PubMed
Brown KJ, Formolo CA, Seol H, Marathi RL, Duguez S, An E, Pillai D, Nazarian J, Rood BR, Hathout Y (2012) Advances in the proteomic investigation of the cell secretome. Expert Rev Proteomics 9:337–345PubMedCentralPubMed
Bujak M, Dobaczewski M, Chatila K, Mendoza LH, Li N, Reddy A, Frangogiannis NG (2008) Interleukin-1 receptor type I signaling critically regulates infarct healing and cardiac remodeling. Am J Pathol 173:57–67PubMedCentralPubMed
Carmeliet P (2005) VEGF as a key mediator of angiogenesis in cancer. Oncology 69:4–10PubMed
Cervio E, Barile L, Moccetti T, Vassalli G (2015) Exosomes for intramyocardial intercellular communication. Stem Cells Int 2015:482171PubMedCentralPubMed
Chen X, Lin X, Zhao J, Shi W, Zhang H, Wang Y, Kan B, Du L, Wang B, Wei Y, Liu Y, Zhao X (2008) A tumor-selective biotherapy with prolonged impact on established metastases based on cytokine gene-engineered MSCs. Mol Ther 16:749–756PubMed
Chen W, Saxena A, Li N, Sun J, Gupta A, Lee DW, Tian Q, Dobaczewski M, Frangogiannis NG (2012) Endogenous IRAK-M attenuates postinfarction remodeling through effects on macrophages and fibroblasts. Arterioscler Thromb Vasc Biol 32:2598–2608PubMedCentralPubMed
Crisostomo PR, Wang Y, Markel TA, Wang M, Lahm T, Meldrum DR (2008) Human mesenchymal stem cells stimulated by TNF-alpha, LPS, or hypoxia produce growth factors by an NF kappa B- but not JNK-dependent mechanism. Am J Physiol Cell Physiol 294:C675–C682PubMed
Das S, Halushka MK (2015) Extracellular vesicle microRNA transfer in cardiovascular disease. Cardiovasc Pathol 24:199–206PubMed
< div class='tao-gold-member'>
Only gold members can continue reading. Log In or Register a > to continue
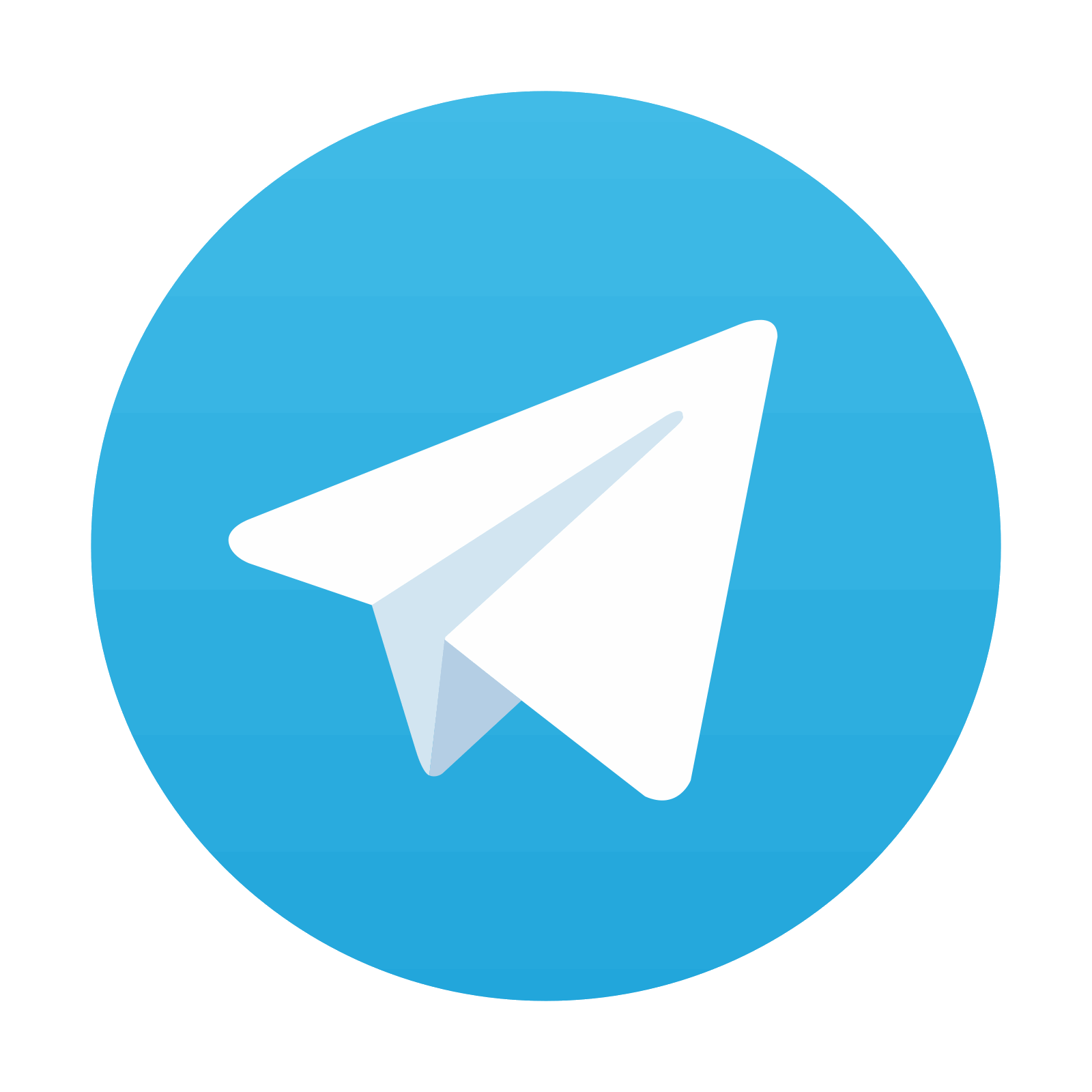
Stay updated, free articles. Join our Telegram channel
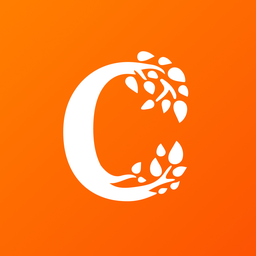
Full access? Get Clinical Tree
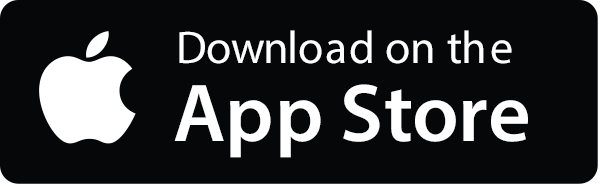
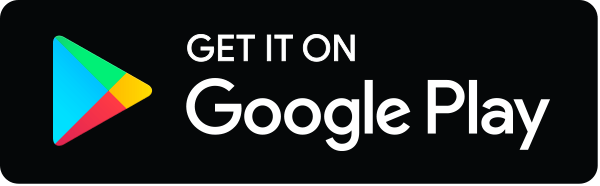