Despite innovations in stent design, including reduction in strut thickness, novel antiproliferative agents, and more recently, the development of bioresorbable polymers, permanent metallic stents remain a nidus for sustained vascular inflammation, in-stent neoatherosclerosis, and impaired coronary vasomotor function. Therefore, the concept of a drug-eluting bioresorbable scaffold (BRS) with properties of medium-term temporary vascular scaffolding and long-term vascular reparative properties is appealing.
Several drug-eluting scaffolds, made of either biodegradable polymers or biocorrodible metals, have been developed and tested in first-in-man (FIM) studies showing comparable performance with current-generation drug-eluting stents (DES) in selected clinical subsets. More recently, large-scale randomized trials have demonstrated noninferiority of BRS compared to current-generation DES with respect to safety and efficacy. This cumulative evidence indicates the gradual maturation of the novel field of vascular reparative therapy.
The impetus for developing drug-eluting BRS was driven by the need for more elastic and conformable platforms instead of stiff and permanent metallic implants to prevent acute and late recoil, seal post-procedural dissections derived from vascular barotrauma, and inhibit in-segment restenosis using appropriate drug elution.1 This technologic endeavor would overcome mid- and long-term effects of rigid metal caging over a previously pulsatile vascular tissue.
As early as 1996, van der Giessen et al2 reported on deploying synthetic polymer-based scaffolds in porcine coronary arteries. His preliminary observations demonstrated marked inflammatory reactions and subsequent intense neointimal proliferation. Further bench studies indicated that lower molecular weight polymers were an important determinant of these adverse vascular responses.2 Current polymeric BRSs are therefore engineered using high-molecular-weight polymers, with poly-L-lactic acid (PLLA) being the most commonly used material. Other polymeric BRSs include tyrosine-derived polycarbonates and poly(anhydride-esters) composed of salicylic acid. Furthermore, an alternative approach to polymeric materials has been the use of magnesium metallic alloys (Table 31-1). Long-term potential advantages of BRS over permanent metal stents have been proposed based on preliminary observations from multicenter registries.
First- and second-generation metallic DESs have been associated with midterm endothelial dysfunction as well as a blunted vasodilatory response to endothelial-independent vasodilators such as nitroglycerine.3,4 Experimental models and clinical studies indicate that both polymeric and magnesium-based BRSs result in improved endothelial function, restoration of non–endothelial-dependent coronary vasoreactivity, and retained vessel pulsatility within and adjacent to the BRS deployed coronary segments.5,6 In addition to the pure biomechanical advantages of a more compliant platform, it is postulated that certain endothelial cell signaling and mechanotransduction pathways may be favorably affected by BRS. Finally, compensatory adaptive expansive remodeling with regression of plaque between the struts and the vessel wall resulting in incremental lumen gain has been described in observational studies.
Wentzel and colleagues first demonstrated, in a porcine model, that alterations of vessel geometry following stenting resulted in unfavorable rheologic profiles in the treated segments. The authors found that permanent metallic stent implantations changed the 3-dimensional coronary geometry at the proximal edge by 121% and the distal edge by 100%, inducing regions of low wall shear stress with subsequent development of neointimal hyperplasia and asymmetric patterns of in-stent restenosis. In contrast, both polymeric- and magnesium-based scaffolds were found to result in improved retention of baseline vascular curvature and angulation, with increased gain over 12 months in the ABSORB Cohort B.5,7 It is hypothesized that such improvement in vascular conformability will translate into more physiologic vascular flow patterns, which, in turn, can facilitate homogenous long-term scaffold healing patterns. These vessel-level differences in post–device deployment flow patterns, as well as strut-level hemodynamic differences, between BRS and permanent metallic stents are being investigated prospectively in ongoing studies.8,9
In-stent neoatherosclerosis has been observed with both metallic DESs and bare metal stents (BMS). The timing of neoatherosclerosis appears to be earlier with DES (6-24 months) compared with BMS (>24 months)10 (Fig. 31-1). The mechanism of neoatherosclerosis is not fully elucidated; however, incomplete endothelial coverage and sustained endothelial dysfunction are thought to play important roles. Although selected cases with neoatherosclerosis following BRS deployment have been published,11 intravascular imaging data from limited but consecutive patient cohorts treated with BRS do not demonstrate evidence of neoatherosclerosis (ABSORB Cohort B). It is hypothesized that the combination of complete scaffold resorption, regenerated intact endothelium with restored vasomotor function, and plaque passivation has the potential to reduce the risk of neoatherosclerosis.12,13
FIGURE 31-1
Distal stent edge neoatherosclerosis 6 years after bare metal stent implantation. A. Distal right coronary artery (RCA); the trilaminar vascular structure is preserved between 1 and 5 o’clock. B. The total circumference of the distal edge appears to be occupied by neoatheroma consistent with a lipid-core plaque (white asterisk). Signs of neovascularization are observed at 1 o’clock. C. Distal edge occlusion as a consequence of plaque growth (white asterisk) at the side of the right ventricular branch takeoff. D. Distal stent edge neoatherosclerosis. The white arrows demonstrate the shadow behind the metallic struts. E-I, K. Metallic struts at different stages of healing. J. Delayed strut healing. AM=acute marginal; GWS=guide wire shadow.

Absorbable scaffolds appear as more appropriate technologies for the treatment of pediatric obstructive vascular lesions such as premature coronary atherosclerosis, aortic coarctation, and pulmonary artery stenosis. Permanent metallic implants limit vessel growth and require future surgical removal, in contrast to BRS, which may allow natural vessel growth after the time of radial strength elimination and further resorption.
There are additional proposed advantages of BRS. First, they provide options for coronary artery bypass surgery in patients with advanced distal disease. This may seem esoteric, but not infrequently patients undergo percutaneous interventions with metallic stents that extend into the distal vessels, limiting targets for future coronary bypass. BRS for treatment of such distal diffuse disease may allow future bypass after resorption of the scaffold. The second advantage is the feasibility of computed tomographic imaging of the coronary arteries. With continued improvements in computed tomography angiography (CTA), including postprocessed computational techniques for hemodynamic lesion assessment, it is anticipated that noninvasive assessment of coronary arteries and bypass grafts will increase.14,15 Presently, coronary segments with metallic stents cannot be visualized by CTA, but segments treated with BRS can be adequately visualized, thereby reducing the need for invasive coronary angiography in patients with prior percutaneous coronary intervention (PCI).16 The third advantage is percutaneous treatment of large bifurcation lesions. The rationale is that one might achieve adequate revascularization with a 2-scaffold technique, with careful lesion preparation and image-guided postdilatation limiting risk of in-stent restenosis and with bioresorption over time not incurring long-term risk of stent thrombosis or neoatherosclerosis.17 The final advantage is a reduction of angina due to the favorable effects of BRS in the microvasculature. The observation that for similar epicardial revascularization, patients treated with BRS had lower rates of angina at follow-up was derived from the 1-year interim analysis of the ABSORB II randomized clinical trial, which compared a BRS with Xience metallic DESs (Abbott Vascular, Santa Clara, CA).18 However, this was not confirmed in the larger ABSORB III clinical trial, and ABSORB IV is currently under way to further evaluate this hypothesis.
Polymers have specific mechanical properties defined by 2 parameters. First is the Young tensile modulus of elasticity, which describes the material’s transient deformation in response to an applied force. The Young modulus quantifies the elastic property or measure of stiffness of the material and is highly dependent on temperature. Second is the tensile strength, which quantifies the amount of stress the material will endure before suffering permanent deformation. The spectrum of the Young tensile modulus of elasticity and tensile strength for polymeric and metallic scaffolds as well as permanent metallic implants is shown in Table 31-2.
Tensile Modulus of Elasticity (GPa) | Tensile Strength (MPa) | Elongation at Break (%) | |
---|---|---|---|
Poly-L-lactic acid | 3.1-3.7 | 60-70 | 6 |
Poly-D,L-lactide | 3.1-3.7 | 45-55 | 6 |
Poly (glycolide) | 6.5-7.0 | 90-110 | 2 |
Cobalt-chromium | 210-235 | 1449 | 40 |
Stainless steel 316L | 193 | 668 | 40+ |
Nitinol | 45 | 700-1100 | 20 |
Magnesium alloy | 40-45 | 220-330 | 20 |
The chemical properties of scaffolds inform the site where degradation begins as well as the rate of degradation. Hydrolytic degradation favors the amorphous tie chains of the polymer that connect the crystal lamellae (Fig. 31-2). Once these tie chains are hydrolyzed, then bulk degradation of the crystal lamellae takes place starting from the inside core of the strut progressing to the strut surface. The surface polymer in which the antiproliferative agent is blended consists of both D (dextro) and L (levo) isomers of polylactic acid (PDLLA). PDLLA is fully amorphous, lacking crystalline structures, which results in relatively rapid degradation.19
FIGURE 31-2
Bench bioresorption of poly-L-lactic acid. A. The pathway of ester bond hydrolysis of poly-L-lactic acid. B. Poly-L-lactic acid is semicrystalline (70% crystallinity) consisting of amorphous tie chains linking the semicrystalline phased polymer. Degradation process starts at the sites of amorphous tie chains. C. Graph showing the change of radial support, molecular weight, and mass loss over time after hydrolysis. The device retains its radial support until 6 months; after this time point, it is transformed to a passive implant with no supportive properties.

The first stage in the process of polymer bioresorption is hydration. Polylactides are relatively hydrophilic; thus water diffuses into the less dense amorphous regions of the implant and hydrolyzes the ester bonds. Random chain scissions occur at this stage, leading to reduction of the polymer’s molecular weight. The second stage is characterized by continuous cleavage of the amorphous tie chains reducing the radial strength of the scaffold, leading to structural discontinuities. During the third stage, polymer chains that have been hydrolyzed to short lengths diffuse out of the implant (mass loss) as they are increasingly hydrophilic and soluble in aqueous solution. Following these sequential stages, oligomeric polylactic acid molecules hydrolyze to lactic acid monomers, which deprotonate (release of a proton [H+]) to lactate. Lactate is converted to pyruvate and enters the citric acid cycle (Krebs cycle) and is further metabolized in carbon dioxide and water excreted through the lungs and kidneys, respectively.20
In vivo polymer bioresorption has been analyzed by histology and visualized with optical coherence tomography (OCT). In the first experimental study that investigated the PLLA-based AbsorbTM bioabsorbable vascular scaffold (BVS) biodegradation process, 35 polymeric scaffolds (3.0 × 12 mm) were implanted in the main coronary arteries of 17 healthy Yucatan minipigs. OCT was performed after the procedure (n = 2) and at 28 days (n = 2), 2 years (n = 3), 3 years (n = 5), and 5 years (n = 5).21 Struts imaged with OCT, had a box-shaped appearance after implantation with sharply defined borders. At 28 days, 82% of the struts visualized with OCT remained box-shaped, whereas by histology, all struts appeared intact with no evidence of resorption. At 2 years, 80% of the struts still had a box-shaped appearance; however, polylactide was not able to be detected by gel permeation chromatography. Histology confirmed that PLLA had been replaced by proteoglycan-rich matrix (glycoconjugate) that stained positive with Alcian blue. At 3 years, only 5% of strut voids were box-shaped imaged with OCT, whereas 44% showed dissolved black box, 35% showed dissolved bright box, and 16% displayed an open box appearance.
Pathologic assessment at this time point showed that connective tissue had replaced the resorption sites previously occupied by proteoglycan-rich matrix. At 4 years histology revealed that strut voids are minimally discernible as foci of hypocellular connective tissue. These observations provide evidence of complete polylactide resorption at 2 years, whereas at 4 years, both in vivo OCT and ex vivo histology confirmed complete integration of the strut voids into the arterial wall.22,23
The Igaki-Tamai (Kyoto Medical Planning Co, Kyoto, Japan) scaffold was the first polymer-based device that underwent FIM evaluation. The material of the scaffold was PLLA, had a helical zig-zag design with sinusoidal hoops linked by 3 connectors, and carried 2 radiopaque cylindrical gold markers at the proximal and distal ends (Fig. 31-3A). The device was mounted over a conventional angioplasty balloon and was self-expanding following heated contrast injections (up to 70°C or 158°F) to inflate the delivery balloon. Importantly, this first BRS was not a drug-eluting device.
FIGURE 31-3
Ten-year follow-up of the Igaki-Tamai scaffold. A. Macroscopic view of the polymeric scaffold demonstrating a helical zig-zag design interconnected with straight bridges. B. Angiographic view of the right coronary artery (RCA) demonstrating a significant mid-RCA lesion before procedure and the excellent angiographic result after implantation of the 2 Igaki-Tamai scaffolds. B’ and B”. Angiographic views 10 years after implantation of the Igaki-Tamai scaffolds without evidence of in-scaffold restenosis. Yellow asterisks demonstrate the gold markers at each proximal and distal scaffold end. Red asterisk demonstrates the outgrowth of a side branch. I-VI. Optical coherence tomographic views of the RCA after 10 years of Igaki-Tamai scaffolding. A smooth lumen with no remnants of strut voids is evident. (Reproduced by permission from Onuma Y, Garg S, Okamura T, et al. EuroIntervention. 2009;5(Suppl F):F109-F111.)

The FIM study was performed in 15 patients (19 lesions, 25 scaffolds implanted) demonstrating absence of major adverse cardiac events (MACE) or scaffold thrombosis defined by angiographic follow-up at 30 days. Longitudinal evaluation at 6 months demonstrated an acceptable angiographic loss index (late loss/acute gain) of 0.48 ± 0.32, which was comparable to BMS. Interestingly, intravascular ultrasound (IVUS) demonstrated scaffold expansion from post-deployment to 6-month follow-up (7.42 ± 1.51 mm2 to 8.13 ± 2.52 mm2), a finding observed for the first time with coronary implantable devices.24 Target lesion revascularization (TLR) rates reached 6.7%, and 4-year MACE-free survival rates were 82%. The long-term clinical follow-up (>10 years) of 84 Igaki-Tamai scaffolds implanted in 63 lesions was recently reported showing a 10-year MACE rate of 50%, with a TLR rate of 38% and 2 cases of scaffold thrombosis25 (Fig. 31-3B).
Although the technology did obtain the Conformité Européenne (CE) mark for peripheral interventions, it failed to do so for coronary revascularization largely due to the need for heat to induce scaffold expansion and the requirement for 8-Fr guide catheters for scaffold deployment.
Magnesium (Mg), the lightest metal, is alloyed with rare earth metals, including aluminum, manganese, lithium, zinc, and zirconium. This class of bioresorbable devices belongs to biocorrodible metals characterized by high corrosion rates (complete biodegradation within 3 months), with end products being elemental Mg and inorganic salts (Fig. 31-4). The earliest proof-of-mechanism that demonstrated the biocompatibility of these materials with vascular tissue was introduced by Heublein et al26 who employed absorbable Mg devices in porcine coronary arteries and demonstrated rapid endothelialization with low inflammatory response.
FIGURE 31-4
Schematic representation of magnesium (Mg)-based scaffold bioresorption. The end product following magnesium scaffold bioresorption facilitated by hydrolysis is amorphous hydroxyapatite. The whole process takes up to 9 months with the first-generation DREAMS scaffold. Drug elution occurs within 3 months after device implantation.

The PROGRESS AMS (Clinical Performance and Angiographic Results of Coronary Stenting With Absorbable Metal Stents) trial was a nonrandomized, multicenter, prospective FIM trial that assessed the safety, efficacy, and performance of the first absorbable Mg stent (AMS-1; BIOTRONIK, Berlin, Germany). The AMS-1 platform was 93% Mg and 7% rare earth metals, the strut thickness was 165 μm. Sixty-three patients with stable coronary artery disease (CAD) were treated with 71 stents. The angiographic in-stent lumen loss was 1.08 ± 0.49 mm at 4 months, and IVUS imaging suggested that most of the struts were fully resorbed, with only strut remnants being visible and fully embedded into the neointimal layer. TLR rates were rather high (24% at 4 months and 45% at 1 year).
Although this study demonstrated safety of AMS-1, with no reported death, myocardial infarction, or stent thrombosis, imaging and clinical results raised concerns over the further use of this generation in coronary interventions as increased neointimal formation and vessel recoil became evident. Subsequently, AMS-2 and AMS-3 were developed to overcome the aforementioned limitations primarily caused by the lack of drug elution and early loss of radial strength.27
AMS-2 provided an improved Mg alloy with higher collapse pressure of 1.5 bar (compared to 0.8 bar with AMS-1), a slower degradation rate with expected absorption after 9 to 12 months, and a reduced strut thickness of 125 μm with rectangular shape to enhance stent integrity. The AMS-3 was designed to address the issue of excessive neointimal proliferation; thus, a bioresorbable matrix for controlled release of paclitaxel was added to the previous AMS-2. The new device was named drug-eluting AMS (DREAMS) 1.0. It was evaluated for safety, feasibility, and efficacy in the prospective, multicenter, FIM BIOSOLVE-I trial (Safety and Performance of the Drug-Eluting Absorbable Metal Scaffold [DREAMS] in Patients With De Novo Coronary Lesions).7 Forty-six patients with stable or unstable CAD or silent ischemia were treated with 47 stents. The angiographic in-stent lumen loss was 0.64 ± 0.50 mm at 6 months and 0.52 ± 0.49 mm at 1 year, which represents a 61% reduction compared to the 4-month results of AMS-1. Serial IVUS imaging confirmed the angiographic observations showing in-scaffold area obstruction of only 6.2% (P < .0001) at 1 year, attributed to neointimal formation with extra-scaffold plaque area increase. The TLR rate reached 7% with no reported episodes of scaffold thrombosis at 3 years of follow-up.
An important part of BIOSOLVE-I trial included the evaluation of anatomic changes induced by the scaffold from before to after the procedure and at follow-up. In particular, vessel curvatures showed a significant change of –32.1% (P < .0001) from before to after the procedure and returned to the pre-implantation levels at the 12-month follow-up.28 Vessel angulation similarly changed significantly by –40.5% (P < .0001) from before to after the procedure (vessel straightening) and increased by 33.1% at 12-month follow-up. These findings reflect the restoration of vessel anatomy after DREAMS scaffold bioresorption, which, from a rheologic perspective, potentially translates into improved flow patterns over the scaffolded segments and the transition zones. The beneficial hemodynamic implications of these conformable materials may minimize the flow-dependent changes of wall shear stress, which precipitate neointimal formation and subsequent silent or clinical restenosis; however, this hypothesis-generating concept remains to be proven by future clinical trials.29
A second-generation DREAMS device has been recently developed that elutes sirolimus, carries 2 tantalum radiopaque markers at both ends, and provides higher bending flexibility and slower resorption rate compared to the previous generation. Preclinical data are encouraging, showing increased endothelialization rates and decreased inflammatory scores, and the BIOSOLVE-II study has been designed to assess the safety, efficacy, and feasibility of this generation in 123 patients, with follow-up investigations scheduled at 1, 6, 12, 24, and 36 months. IVUS, OCT, and vasomotion testing were completed in a subset of patients at 6 months. The primary end point of in-segment late lumen loss at 6 months was 0.27 ± 0.37 mm. The 2nd generation DREAMS scaffold aka Magmaris received CE mark regulatory approval in 2016.
Current evidence suggests that biocorrodible metallic scaffolds can be safely implanted in patients with favorable clinical outcomes. Despite the encouraging preliminary observations, further randomized clinical trials with head-to-head comparisons with newer generation DESs will define whether these technologies will be adopted over contemporary metallic stents.30
The first-generation Absorb BVS (Abbott Vascular) was Absorb BVS 1.0 (Fig. 31-5). The device had circumferential out-of-phase sinusoidal hoops linked either directly or by straight polymeric bridges. The strut thickness including the polymer drug coating was 156 μm, and the crossing profile of 1.4 mm (crimped stage) was slightly larger compared to that of contemporary metallic stents. The device had to be kept refrigerated at –20°C to prevent early aging because room temperature is a precipitating factor for polymer cracking during scaffold deployment.20 The Absorb BVS 1.0 was evaluated in 30 patients with simple de novo native coronary artery stenosis for safety, feasibility, and efficacy in the ABSORB Cohort A. Follow-up included invasive angiography, IVUS, virtual histology IVUS (VH-IVUS), and OCT at 6 months and 2 years, and clinical end points were assessed at 6 months and 1 and 2 years. Noninvasive coronary angiography with multislice computed tomography was also performed at 18 months and 5 years.
FIGURE 31-5
Multi-imaging assessment of the AbsorbTM bioresorbable vascular scaffold (BVS). The Absorb BVS 1.0 was the first-generation device used in the ABSORB Cohort A trial. The second-generation Absorb BVS 1.1 has been redesigned and is currently under clinical use. Intravascular imaging modalities such as intravascular ultrasound (IVUS), virtual histology IVUS (VH-IVUS), and optical coherence tomography (OCT) have provided important insights in the evaluation of bioresorbable scaffolds. The top panel of cross-sections shows IVUS, VH-IVUS, and OCT following Absorb BVS implantation, whereas the bottom panel shows 6-month follow-up. Polymeric materials, as opposed to metal materials, do not reflect sound or light; thus, shadow behind the struts is not visible. Guide wires and platinum markers are the only components that generate shadow over the circumference of the cross-section. VH-IVUS detects polymeric struts as a dense calcium tissue component similar to metal stents; in contrast to metal stents, this unique property is a surrogate marker of bioresorption with biodegradable scaffolds.

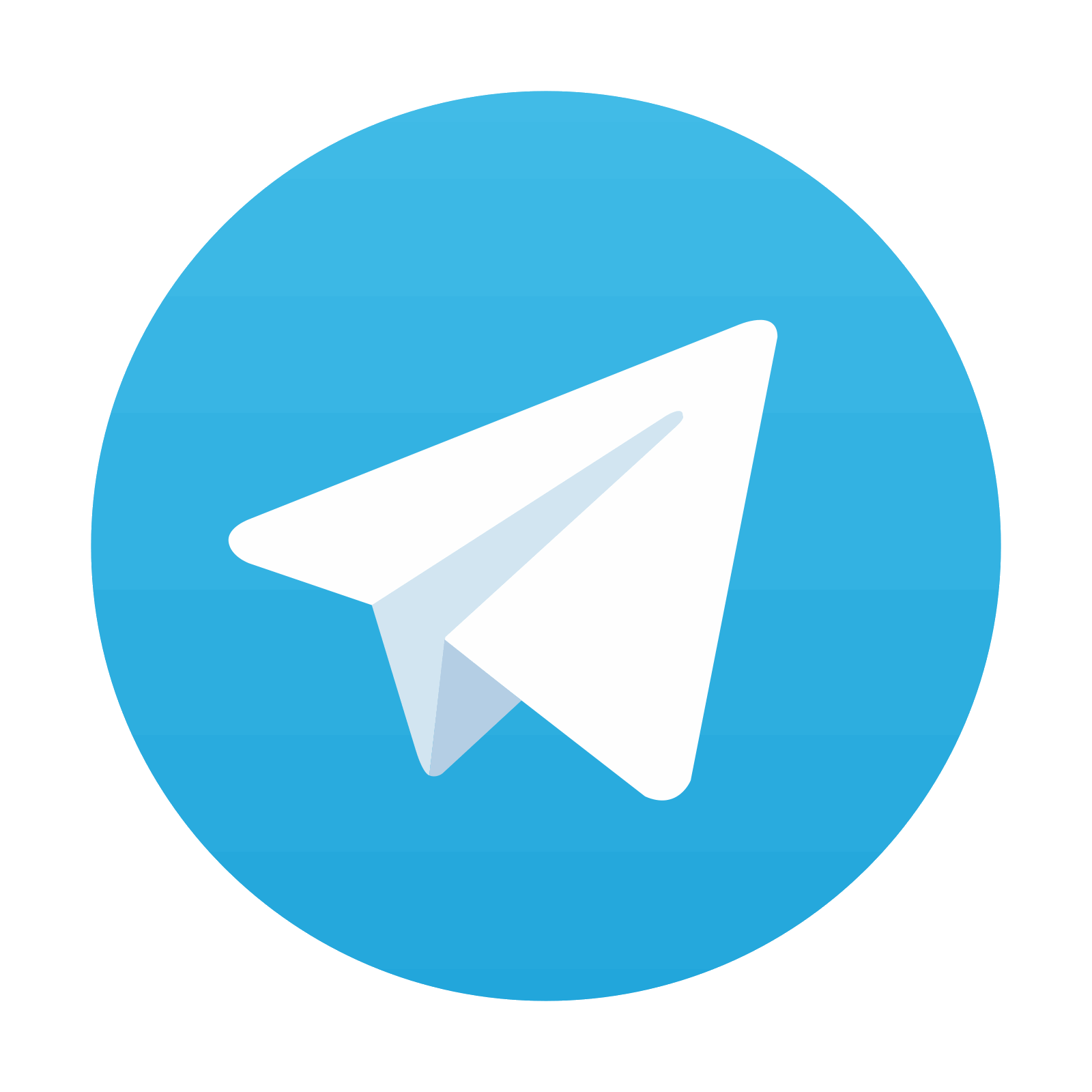
Stay updated, free articles. Join our Telegram channel
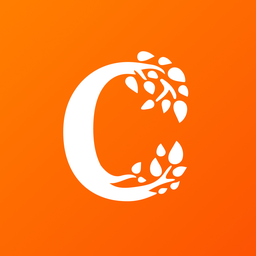
Full access? Get Clinical Tree
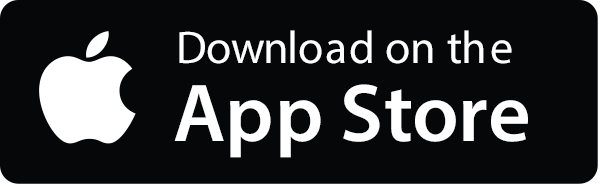
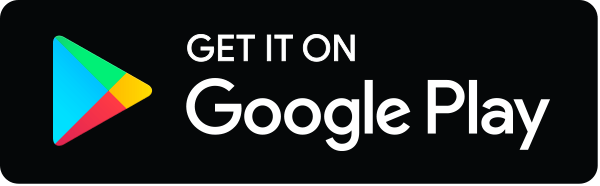
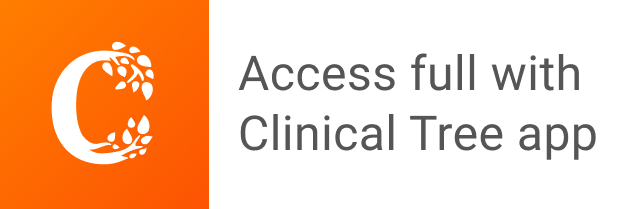