Fig. 15.1
Alterations in endothelial cell function and phenotype as determined by environmental hemodynamics. (a) Endothelial cell responses to high shear stress. (b) Endothelial cell responses to low shear stress
Normal laminar shear stress is critical in maintaining normal physiologic vascular function including thromboresistance, barrier function, and vascular tone. In contrast, low or oscillary shear stress results in disturbed flow conditions and plays an important role in atherogenesis and bypass failure [13] (Fig. 15.1). Work done by Gimbrone and colleagues has demonstrated the relationship between decreased shear stress and atherogenesis [14]. Decreases in shear stress are accompanied by disturbances in normal endothelial cell function, and repair mechanisms within the endothelium are reduced along with the production of endothelial nitric oxide synthase (eNOS). Moreover, systemic risk factors such as hypertension and hyperlipidemia exacerbate endothelial intrinsic dysfunction. On a cellular level, this results in increased production of reactive oxygen species (ROS), altered lipoprotein permeability, leukocyte adhesion, and cellular proliferation [15].
Hypoxic Physiology
Hypoxia, depletion of the circulating oxygen content, is recognized as the driving force behind ischemic injury and, on a cellular level, endothelial cells respond to this insult by undergoing the phenotypic change now universally recognized as endothelial cell activation. This results in a series of alterations including the release of stored inflammatory mediators, changes in endothelial cell surface protein expression, and the conversion from aerobic metabolism to anaerobic alternative pathways [16]. The vascular endothelium is responsible for regulating membrane permeability, vascular tone, coagulation, and inflammation, and it does so by dynamically responding to any systemic alterations, such as hypoxia, within the vascular environment [17]. Yet, despite its wide range of adaptability, the vascular endothelium likewise remains very sensitive to hypoxic insults, inflammatory stimuli, and physical injury from surgical manipulation or hemodynamic stress, and it is this interplay between adaptation and injury that makes cardiovascular surgery a challenging paradox [16] (Fig. 15.2).
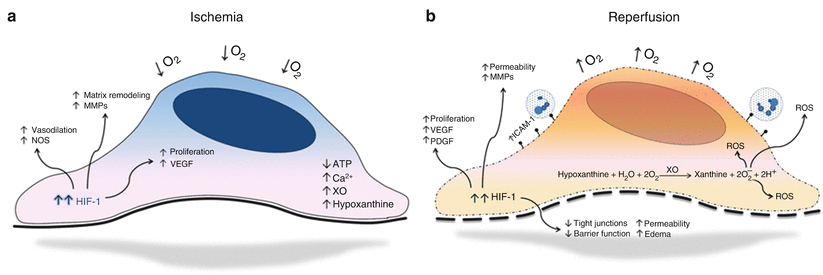
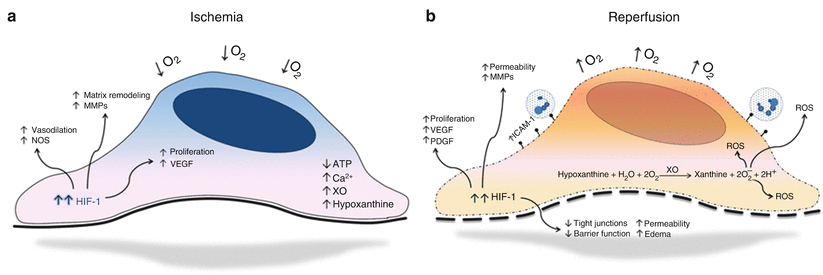
Fig. 15.2
Endothelial cell responses to ischemia. (a) Endothelial cell hypoxic physiology. (b) Endothelial cell responses to reperfusion
In contrast to other cell types, the vascular endothelium is comparatively resistant to decreases in oxygen content [17]. Under ischemic conditions, aerobic cellular energy depletion leads to an atypical accumulation of cytoplasmic metabolites and failure of oxygen-dependent membrane transport systems. Of most significance is the documented intracellular increase in calcium ion concentration and the upregulation of xanthine oxidase (XO) transcription and synthesis. Although restoration of blood flow after prolonged ischemia is essential for possible physiologic salvage, reperfusion itself exacerbates endothelial cell injury. XO, an endothelial cell-associated enzyme, increases under hypoxic conditions and, with the restoration of blood flow and oxygen, leads to the intracellular buildup of reactive oxygen species (ROS) [18]. Once produced, ROS cause localized and systemic cellular damage through both direct injury to membranes and proteins and indirectly through activation of proapoptotic pathways [19]. This reperfusion damage is further augmented as damaged cellular membranes are exposed to a replenished intravascular supply of calcium. Acting as a second messenger, calcium triggers activation of various enzymes crucial to the production of proinflammatory mediators [20].
Under normal conditions endothelial cells form an overlapping monolayer that permits only controlled passage of molecules. This monolayer is further enhanced through the presence of tight junctions that reinforce endothelial cell barrier function. Hypoxic conditions lead to alterations in endothelial cell barrier function and vessel permeability. In response to decreased oxygen content, there is loss of tight junctions between adjacent endothelial cells leading to gap formation and increased permeability between cells. These changes are likewise accompanied by decreases in intracellular cyclic AMP, which is essential for maintenance of the actin-based cytoskeletal architecture and normal barrier function [4, 21]. This increased permeability results in what is clinically observed as ischemia reperfusion edema.
Hypoxic adaptation in endothelium leads to the transcriptional induction of a series of genes that participate in angiogenesis, metabolism, and cell proliferation, and the primary mediator of this response is hypoxia-inducible factor-1 (HIF-1), an oxygen-sensitive transcriptional activator [22]. The HIF-1 heterodimeric protein consists of two subunits, a beta subunit that is constitutively expressed (HIF-1β) and an oxygen-regulated alpha unit (HIF-1α). The stability and activity of the alpha subunit are regulated by its post-translational modifications, and, under normoxic conditions, this subunit is degraded. Conversely, in hypoxia, the beta subunit becomes stable resulting in the regulation of target gene expression [22]. Previous studies have demonstrated that HIF-1 plays a critical role in endothelial angiogenesis through both paracrine and autocrine mechanisms [23, 24]. Recent discoveries have shown that hypoxia-activated HIFs induce endothelial expression of several critical angiogenic factors, including vascular endothelial growth factor (VEGF), nitric oxide synthase (NOS), platelet-derived growth factor (PDGF), Ang2, and others [25]. HIFs have also been found to be mediators of endothelial survival pathways where hypoxic upregulation results in cellular proliferation. Clinically, this can result in a wide spectrum of remodeling, including angiogenesis and neointimal hyperplasia (Fig. 15.2).
Shear Stress and Arteriogenesis
Study of arteriogenesis, the enlargement of preexisting arterial connections into true collateral arteries, began in the 1700s with the work of Albrecht von Haller, a Swiss anatomist, who dissected human hearts and found that coronary arteries provide a system of interarterially connected vessels on the side of high arterial pressure. His findings suggested that these conduits were functional arteries, larger in size than capillaries [26]. Today, this process is recognized as arteriogenesis, and, unlike angiogenesis, which is primarily mediated by hypoxia, initiation of collateral artery growth and remodeling is dependent on alterations in hemodynamic forces. Circumferential wall stress and fluid shear stress are recognized and the two primary forces responsible for this complex process; however, uncertainties continue with regard to the specific contributions and overall importance of either toward arteriogenesis. Some uncertainty comes from the observation that fluid shear stress, with a typical range of 20–30 dyn/cm2, is a weak force when compared with circumferential wall stress, which is 106 times greater [27].
In the early 1920s, Murray proposed that the vascular system’s branching configuration exists so as to minimize the amount of mechanical and metabolic work to provide adequate blood flow. Using this model, it can be surmised that fluid shear stress remains constant throughout the vasculature and that blood flow is proportional to the cube of each individual vessel’s diameter. Additionally, shear stress remains proportional to blood flow and inversely related to the cube of the radius [27, 28]. Accordingly, it is hypothesized that shear stress regulates the acute early phase of arteriogenesis where small increases in collateral artery diameter result in significant decreases in shear stress [29]. The formation of collateral circulation after an arterial occlusion correlates well with the observed increase in shear stress where increased collateral flow is caused by the pressure redistribution from pre-existent, now occluded, vessels [30]. It is also recognized that increases in collateral diameter end once shear stress normalizes. Interestingly, despite early and abrupt normalization, cellular remodeling of collaterals persists beyond this acute period, and a shift from quiescence toward proliferation is observed in both endothelial and vascular smooth muscle cells [31]. As a result, it has been proposed that circumferential wall stress, which remains elevated, is the more dominant force in this later stage of arteriogenesis. Regardless of the dominant mediators, collateral remodeling frequently reaches a plateau, at which point hemodynamic alterations are unable to compensate for progressive atherogenesis. Indeed, when these compensatory systems fail or are overwhelmed, what follows next is the clinical entity of critical limb ischemia.
Surgical Solutions for CLI
Autologous Vein Grafts
Today, autologous vein grafting is the recognized standard for infrainguinal arterial revascularization, yet prior to the pioneering work done in the early 1900s, the predominating belief was that repair of major vasculature was beyond the capacity of surgical technique. Unconvinced that the 1894 penetrating portal venous injury of French president Carnot was not treatable, Carrel would undertake the task of refining vessel anastomosis. He would go on to establish the modern fundamentals of bypass surgery with his combined techniques of delicate vessel handling, fine suture material, and triangulation [21]. The future of bypass surgery would then be further progressed by Kunlin, who in 1948 would be the first to successfully use a reversed saphenous vein graft in the treatment of lower extremity ischemia [32]. In 1962, Sabiston would then go on to use a modification of this technique in what we recognize today as the modern coronary bypass. Interestingly, although much has changed from the pioneering days of surgical vascular research, what remains is that the conduit of choice for lower extremity bypass continues to be the greater saphenous vein.
Physiology of Vein Graft Adaptation
Venous anatomy echoes arterial, and the intima, media, and adventitia are easily identified as the major anatomic vessel wall components. It is well known that, unlike arteries, veins have a reduced medial component, which results in a thinner vessel wall and typically allows for increased compliance. From prior work, it is known that vein compliance is not a static property, but rather dependent on its environment. Although it is commonly assumed that native veins are more compliant than arteries, in actuality, this increased compliance only remains present up to pressures of 35–50 mmHg [33]. Under typical arterial pressures, vein grafts become stiff and lose compliance, while matched arteries remain moderately distensible. Accordingly, a more accurate description is that veins are more compliant than arteries at low pressures, but less compliant than arteries when subjected to arterial pressures.
Low pressure and low flow are the dominant hemodynamic entities in the normal venous environment, and, unlike the physiology observed in arterial circulation, veins are not subjected to pulsatility, high flow, or high pressures. All vessels, venous and arterial, are subject to mechanical forces in the form of shear and circumferential wall stress. Effects of circumferential strain directly correlate with blood pressure, and in the venous system, these maximal pressures are understandably reduced. The circumferential venous forces that remain go on to be counteracted by the entirety of the vessel wall, and the cellular components in the intima, media, and adventitia are uniformly affected. Conversely, shear stress, the frictional force of blood along the intima is distinctive in that it exerts its force primarily on endothelial cells. Fluid shear stress likewise displays significant variation between venous and arterial systems, and prior studies have found 1–6 dyn/cm2 to be typical of venous shear stress. This is in large contrast to arterial vasculature where anywhere from 10 to 70 dyn/cm2 is the prevailing norm [11]. This abrupt hemodynamic alteration has been implicated as the mechanism responsible for initial endothelial desquamation, which is observed to occur in the first week of graft placement. In such situations, graft endothelium regeneration appears to occur quickly with complete healing observed in some veins at 48 h [34]. Nonetheless, concerns regarding the long-term effects of acute arterial disruption of venous endothelium remain with respect to graft patency.
Indeed, long-term patency for autologous bypass is largely dependent on how successfully venous conduits adapt to arterialization. As first suggested by Owens et al., the process of successful vein graft adaptation appears to involve at least two distinct temporal phases: early outward remodeling of the lumen and delayed acquisition of wall stiffness [35]. In 2006, in a prospective human study, the authors showed that 72 % of venous grafts dilate during the first post-surgical month and that no appreciable changes occur beyond this time. During the first 6 months, a nearly 40 % increase in conduit stiffness was found with the greatest relative increase occurring during the initial first 3 postoperative months. Clinically, grafts that demonstrated early positive remodeling in the form of lumen dilatation appeared to have increased primary patency, and a trend toward greater wall stiffness at 1 month was noted in grafts that failed [35]. Further work done by the same authors in 2008 underscored the critical role of systemic inflammation in vein graft remodeling. In this study, there was positive correlation noted between vein graft diameter and initial shear stress. This shear-dependent response, however, was disrupted in patients with an elevated baseline high-sensitivity C-reactive protein (hsCRP). Moreover, despite similar vein diameter and shear stress at implantation, grafts in the elevated hsCRP group demonstrated less positive remodeling within the first month and likewise had a propensity to be stiffer [36]. Accordingly, although intricacies and specific mediators remain to be identified, early positive adaptation of vein grafts lends itself to successful long-term patency, and the importance of controlling systemic inflammation deserves emphasis.
Responses of Venous Endothelial Cells to Shear Stress
Hemodynamic shear stress, possibly the most influential component of vascular remodeling and pathology, is the frictional force created by the flow of blood in relation to the luminal vessel wall and endothelial surface. It is in this regard that the creation of a surgical bypass, using a venous conduit, becomes one of the most dynamic processes responsible for endothelial remodeling. Although the technicalities of performing a successful bypass are well established, much of the final outcome hinges on how successful the venous conduit will be in adapting to the high-pressure pulsatile flow arterial circulation where it is acutely placed [37]. Much remains to be learned about the underlying mechanisms responsible for both successful as well as pathologic endothelial adaptation. At present, the majority of our insight into endothelial response to hemodynamics comes from animal experiments in which shear stress is acutely or chronically altered [11].
Classically, increases in shear stress, both acute and chronic, are observed to result in upregulation of endothelial nitric oxide synthase (eNOS) mRNA and protein production [38]. Such increases in eNOS activity result in vessel lumen dilation. Studies investigating this role for nitric oxide (NO) have shown that through the use of L-NAME, a nitric oxide synthase inhibitor, flow-induced vessel dilation is inhibited [39]. In 1997, Clowes and colleagues demonstrated an important relationship between shear stress and neointimal hyperplasia. Using a primate model, the authors inserted PTFE grafts into aortoiliac circulation bilaterally. A neointima was allowed to develop for 2 months, and, at that time, half of the animals were killed. For the remaining group, graft flow was increased through the creation of a femoral arteriovenous fistula. These animals were then killed 2 months post-fistula creation. The findings from this study showed regression of the neointima in those grafts exposed to the additional 2 months of high shear stress. From prior work, using this same model, the authors were also able to show that high shear stress inhibits neointimal growth [39].
Work examining the effects of high and low flow on graft adaptation have shown increased smooth muscle cell proliferation in grafts exposed to low shear stress, and similar findings have also been observed in endothelial cells [40]. In a rat model, where unilateral ligation of the internal and external carotid arteries results in the creation of high- and low-flow arteries, increased endothelial cell proliferation is seen at 24, 48, and 72 h post-ligation in the reduced flow carotids. With this model, the achieved alteration in flow reflected true arterial and venous circulation, with a comparative shear stress of 33.4 ± 1.1 dyn/cm2 in the high-flow carotid versus 1.4 ± 0.2 dyn/cm2 in the ligated, low-flow complement [41]. These correlations between shear stress and neointimal remodeling provide insight into the mechanisms involved in venous bypass and likewise demonstrate the paradoxical effects that high flow and shear stress can exert on endothelial homeostasis.
Graft Characteristics and Long-Term Patency
Using the best available autologous conduit is universally accepted as the fitting approach for peripheral bypass surgery, and the conduit of choice is the ipsilateral, single-‑segment greater saphenous vein secondary to its documented patency in lower extremity bypass [42]. Other characteristics that define the best autologous conduit however continue to be debated, and much of the data available comes from single-institution retrospective studies that attempted to define meaningful graft characteristics in regard to long-term patency. Traditionally, three surgical configurations, reversed, nonreversed, and in situ, have been accepted for use in infrainguinal vascular bypass work, and each is believed to confer different effects on endothelial cells.
Reversed Vein Grafts
Vein excision with reversal of anatomical orientation and maintenance of intact valves is the essence of a reversed vein grafting technique. Considered to be somewhat basic, usage of reversed vein grafts is typically well adapted for most surgical bypass settings. The reversed technique eliminates the need for valve lysis and thus limits intraluminal manipulation. Concerns with regard to the altered flow dynamic in reversed vein grafts have come from studies showing intraluminal turbulence secondary to retained valves. The surgical creation of a reversed vein graft introduces valves into the arterial system and, by doing so, adds a potential source of turbulence. Such deviations from laminar flow hold the potential to initiate pathological endothelial cell activation. Despite having a reversed anatomy, venous valves do not lie flush with the graft wall, and once subjected to pulsatile arterial flow, these valves are observed to close during diastole [43]. Coupled with the cardiac cycle, valves have the potential to modify flow dynamics by becoming active and preventing the natural arterial backflow, and it has been speculated that midgraft stenotic lesions occur secondary to retained valve leaflets [44]. Other surgical concerns arise from the stasis observed within the valve cusps and the potential for them to function as a thrombogenic nidus [43].
Nonreversed Vein Grafts
In nonreversed technique, the vein is excised, its valves are subjected to mechanical lysis, and it is then oriented in its original nonreversed configuration. Observed advantages come from the preservation of a nonreversed orientation, thus improving the circumferential accord of the arterial graft anastomosis. Intraluminal valve lysis, however, is a requisite and results in endothelial cell trauma and denudation. Venous valve lysis results in significant endothelial trauma, and, from prior work, it is known that the preservation of endothelial cell integrity in venous grafts prevents subsequent morphologic changes, namely thickening of the venous intima and media in response to arterial dynamics [45]. Remarkably, despite the inherent need for more manipulation and handling with nonreversed grafts, improved hemodynamic flow is the resulting outcome following valve lysis. Studies have shown that lysis of valves increases graft flow rates anywhere from 30 to 60 % as compared to grafts with intact valves [46, 47]. Clinically, the improved bypass hemodynamics, shown through higher shear stress and flow velocity, are atheroprotective and correlate with increased long-term graft patency.
In Situ Vein Grafts
The initial concept of utilizing an autologous vein graft while minimizing surgical manipulation is attributed to the work of both Rob and Hall in the early 1960s. At that time, advanced valvulotomes had yet to be developed, and valve lysis was achieved through serial transverse venotomies and individual valve excision [48]. The modern in situ vein graft is created through limited mobilization of both the proximal and distal vein segments, thus allowing them to be used in arterial bypass but simultaneously reducing total vein manipulation and damage to the outer vessel layers, including the vasa vasorum, during harvesting. Some studies have suggested improved patency outcomes in vein bypasses harvested en-bloc with surrounding tissue where venous spasm is believed to be reduced [49]. Prior studies have also demonstrated that the disruption of the vasa vasorum results in an acute decrease in vessel distensibility as well as long-term structural changes and delayed deterioration of vessel elastic properties [50]. Based on such evidence, it has been proposed that by diminishing spasm, the need for high pressure distention, a known mediator of vein wall and endothelial damage, is reduced [49]. There is also evidence to suggest an inverse relationship between vasa vasorum preservation and neointimal hyperplasia. Findings by Gossl et al. showed that vessel areas with diminished vasa vasorum density were more susceptible to hypoxia, oxidative stress, and microinflammation, all factors known to potentiate early atherogenesis [51]. Accordingly, there is modest evidence present that the use of atraumatic techniques, which minimize damage to the adventitia and the vasa vasorum, improve bypass patency.
Although there have been many studies, mainly single center and retrospective, that have attempted to address aspects of surgical technique in relation to lower extremity bypass outcomes, the exact contribution of specific technical factors remains to be fully defined. The most comprehensive data available come from the 2006 Project of Ex-Vivo Vein Graft Engineering via Transfection III (PREVENT III) Study, which was a randomized, double-blinded, placebo-controlled trial of edifoligide for prevention of vein graft failure in patients undergoing lower extremity revascularization for critical limb ischemia. Although the primary and secondary endpoints for PREVENT III did little to modify current medical strategies for limb revascularization, the study was able to provide perhaps the most comprehensive multicenter data available to date with regard to long-debated conduit and technique characteristics, such as vessel diameter, graft type, and conduit orientation [52, 53].
Results for early 30-day graft failure identified several significant technical predictors of early loss of primary patency. Small-conduit diameter was identified as one such technical factor, and primary patency in grafts greater than 3.5 mm was observed to be 93.2 % vs. 85.7 % in grafts with diameters less than 3.0 mm. Not surprisingly, composite grafts showed worse outcomes when compared to single-segment grafts with a primary patency of 84.1 % vs. 92.2 % in non-spliced greater saphenous vein. Bypasses originating from a more distal location showed improved short-term outcomes. Grafts that originated from the popliteal artery had primary patency rates of 93.9 % as compared to those with more proximal anastomoses, such as the common or superficial femoral arteries, where rates were 91.7 % and 87.7 %, respectively. Graft length and the site of distal anastomosis were not found to be predictors of primary patency loss. Although a slight difference was observed between the early failure rates of reversed and nonreversed grafts, 91.6 % patency as compared to 93.3 %, this finding was not statistically significant [53]. At 1 year, graft patency was again found to be detrimentally affected by small conduit diameter with primary patency observed to be 68.4 % in grafts with diameters greater than 3.5 mm vs. 42.4 % for those less than 3.0 mm. Poorer outcomes were observed in bypasses composed of composite spliced vein, those greater than 60 cm in length, and more proximal bypass origination. Moreover, at 1 year, the primary patency rates for reversed (65.0 %) and nonreversed (63.3 %) orientation grafts were equivalent and not statistically significant. From these data, significant identifiable technical predictors of early and late graft failure include use of a small conduit diameter or a composite vein; however, surgical variation with the reversed and nonreversed technique does not appear to be a primary factor in bypass success [53].
< div class='tao-gold-member'>
Only gold members can continue reading. Log In or Register a > to continue
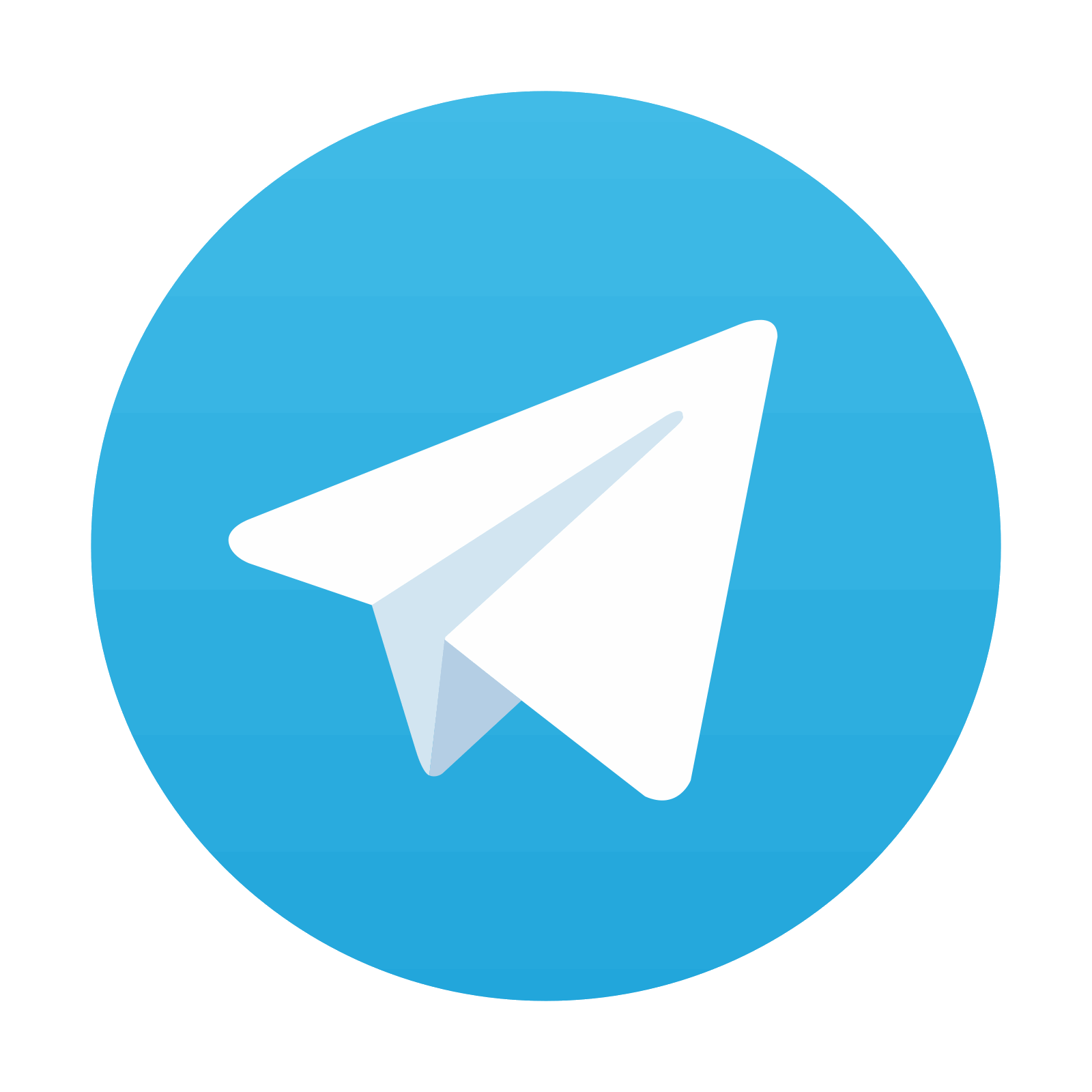
Stay updated, free articles. Join our Telegram channel
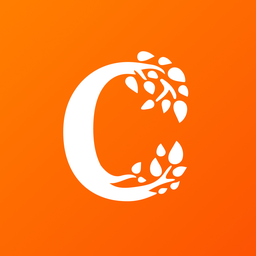
Full access? Get Clinical Tree
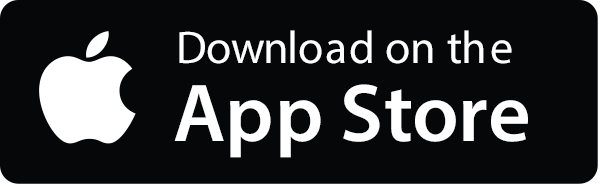
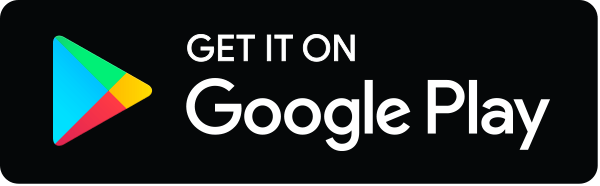