Fig. 1.1
Fluctuations in ovarian and anterior pituitary hormones during a 28-day menstrual cycle. During the follicular phase (day 0–14), estradiol increases. In the end of the follicular phase, estradiol drops while luteinizing hormone (LH) and follicle-stimulating hormone (FSH) rise. This is when ovulation occurs. The following phase is the luteal phase when anterior pituitary hormones (LH and FSH) drop; progesterone rises and then drops, starting the next cycle
Other physiological changes also occur during the various phases of the menstrual cycle. Body temperature is elevated mid-cycle primarily due to the action of progesterone, which increases body temperature approximately 0.2–0.3 °C [1]. Studies investigating how these physiological changes affect sports performance are sparse and inconclusive; however, Lebrun [1] reported variations in vascular volume dynamics, thermoregulation, substrate metabolism, and of primary focus in this chapter, pulmonary function [2].
It is well documented that circulating hormone levels affect pulmonary function. Progesterone may affect hyperventilation [3], partially compensated respiratory alkalosis [4], cause increases in hypercapnic and hypoxic ventilatory response [3, 5], and increase central ventilatory drive, all of which may affect breathing responsiveness during exercise [6]. Additionally, augmented ventilatory drive with reduced airway diameter contributes to expiratory flow limitation during exercise [7] (see section “Expiratory Flow Limitation”). Heightened ventilatory response to exercise may also be due to mast cells in the airways with progesterone and estrogen receptors, which may account for differences in ventilation (V E) [8].
There are many discrepancies in the literature investigating resting ventilation (V E) across the menstrual cycle. Several of these studies report resting V E is elevated in the luteal cycle and tidal volume may be higher (by approximately 100 mL) and end-partial pressure of carbon dioxide (CO2) lower (by about 2–3 mmHg) [4, 9, 10], albeit conflicting evidence suggests no difference in resting V E [11]. As estrogen levels increase, fluid retention and subsequent increases in pulmonary capillary blood volume could affect gas exchange in the lung [12]. Previous work has suggested resting diffusion capacity (DLCO) is reduced during early follicular phase [13], although this finding is not consistent among studies.
Morphology
The structure of the pulmonary system (e.g., lung, airways, and chest wall) is generally considered overbuilt for the exercise demands at sea level [14], although differences in lung morphology exist between men and women. Demands placed on the pulmonary system include increases in minute ventilation and subsequent increases in flow rates, increasing lung volumes to maintain gas exchange of oxygen and carbon dioxide [14]. The structure of the lung is considered to be exceedingly capable of meeting these demands, albeit women are considered to be one of several exceptions. It is possible that the demands of the pulmonary system may exceed its total capacity. This is primarily due to morphological differences in lung size and airway size in females.
Women have smaller lungs and airways compared to men of the same sitting height [15, 16]. Additionally, women have decreased lung volumes and expiratory flow rates compared to height-matched men [17]. Women also have less alveolar surface area and smaller alveoli, consequently decreasing lung diffusion capacity compared to men [15–17]. There are also no sex differences in the recoil properties of the lung [18] or chest wall and pulmonary compliance [19]. Diameter of the trachea is also smaller in women compared to men [20]. In comparing airway size to lung size, women typically have smaller airways relative to lung size [15]. The comparison of airway size relative to lung size is called the dysanapsis ratio (DR) and is classified by Mead [15] using the following ratio:
where FEF50 is forced expiratory flow at 50 %, FVC is forced vital capacity, and P st(1)50 % is lung static recoil pressure at 50 %. Mead was the first to measure the DR of men and women. There was a lower DR in women compared to men, indicating the airways are smaller for a particular lung volume. This has been confirmed by Sheel and colleagues [22], who used tomographic imaging to measure absolute airway size in women. Researchers reported women had significantly smaller airways compared to male ex-smokers matched for lung size .

Pulmonary Function During Exercise
Pulmonary Gas Exchange
It has been previously believed that pulmonary gas exchange does not limit submaximal or maximal exercise at sea level. However, recent studies have shown that endurance-trained men and women experience pulmonary gas exchange impairments during exercise. Generally, healthy men do not experience significant reductions in pulmonary gas exchange during maximal exercise. On the other hand, women are more likely to exhibit pulmonary gas impairments because of morphological differences in pulmonary structures. The efficiency of pulmonary gas exchange is determined by the difference (A-aDO2) between the partial pressure of oxygen in the alveoli (PA) and the arterial blood (Pa). The widening of the A-aDO2 is indicative of pulmonary gas exchange impairments. Mild gas exchange impairment is defined as a A-aDO2 of 25–35 mmHg and a severe pulmonary gas exchange impairment is defined as a A-aDO2 of >35–40 mmHg [23]. The A-aDO2 can be widened by shunts, ventilation–perfusion mismatch (V/Q mismatch), and diffusion limitation (discussed below).
Gas Exchange at Rest and During Exercise
In resting conditions, there is a minor A-aDO2 of ~5–10 mmHg due to V/Q mismatch and shunts. Figure 1.2 displays the arterial PO2 (PaO2), arterial PCO2 (PaCO2), and A-aDO2 during an incremental exercise test. At rest and during submaximal exercise, PaO2 is maintained near resting values. PaCO2 is maintained near resting values as well until alveolar ventilation increases out of proportion to CO2 production to assist in acid–base balance which will lead to a hyperventilatory response and a reduced PaCO2 compared to resting levels. Because alveolar ventilation increases in proportion to exercise intensity, A-aDO2 also increases with increasing exercise intensity. Although A-aDO2 is increasing, PaO2 is maintained near resting levels and PAO2 will increase leading to a greater diffusion gradient between the alveoli and the pulmonary capillary. At maximal exercise, PaCO2 is lower than resting values and PaO2 is maintained near resting values despite the large widening of the A-aDO2. However, in endurance-trained men and women, PaO2 can sometime decrease below resting values which reduces oxygen transport and exercise tolerance and increases exercise-induced peripheral fatigue [24–28].
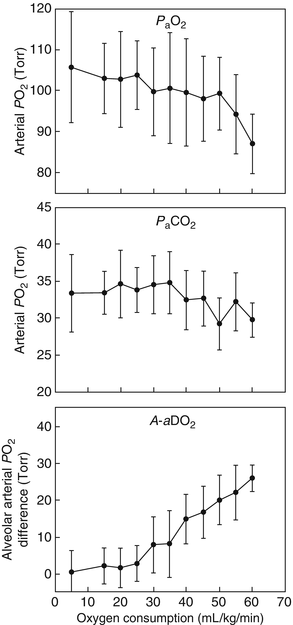
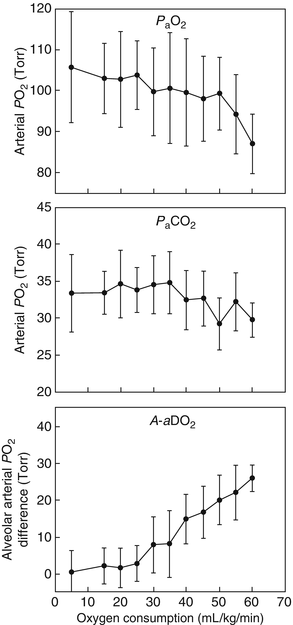
Fig. 1.2
Pulmonary gas exchange during incremental exercise . Arterial PO2 (PAO2) (top panel), arterial CO2 (PACO2) (middle panel), and alveolar–arterial PO2 difference (A-aO2) from rest to maximal exercise. With increasing exercise intensity, PAO2 and PACO2 are maintained near resting values, but A-aO2 increases linearly. At near maximal workloads, PACO2 will decrease due to hyperventilation and PAO2 is generally maintained near resting values except for certain populations (e.g., endurance-trained men and women)
Exercise-Induced Arterial Hypoxemia
Exercise-induced arterial hypoxemia (EIAH) is the reduction in PaO2 and/or oxygen saturated hemoglobin in the arterial circulation (SaO2) during exercise . The adequacy of systemic oxygen transport is characterized by SaO2. The severity of EIAH is defined by the reduction in SaO2 with mild EIAH as 93 % to 95 %, moderate EIAH as 88 % to 93 %, and severe EIAH as <88 % [23]. The widening of the A-aDO2 (due ventilation–perfusion mismatch, shunts, and diffusion limitation), VO2max, and inadequate hyperventilation contribute to EIAH. It is currently thought that the widening of the A-aDO2 contributes 35 %, VO2max 25 %, and inadequate hyperventilation 60 % to EIAH [23]. EIAH is rarely seen in healthy untrained men exercising at sea level. In 1984, Dempsey and colleagues measured arterial blood gases during an incremental exercise test in endurance-trained men. Twelve of 16 men experienced EIAH during submaximal and maximal exercise [25]. The prevalence of EIAH is ~50 % in endurance-trained men during maximal exercise [29]. On the other hand, the prevalence of EIAH in women during maximal exercise is considerably higher (~65–75 %) compared to men [30–32]. Both untrained and endurance-trained women experience EIAH during submaximal and maximal exercise [30, 31]. What are possible mechanisms that explain this increased prevalence of EIAH in women? Although intrapulmonary shunts contribute to the widening of the A-aDO2 during exercise, there are no sex differences in the opening of the intrapulmonary shunts during exercise [33]. Additionally, sex differences do not exist in V/Q mismatch during exercise [34]. However, the higher prevalence of ventilatory constraints (i.e., expiratory flow limitation) [35] and lower lung diffusion surface [16, 17] of women compared to men likely contribute to their higher prevalence of EIAH.
Ventilation–Perfusion Mismatch
Due to the gravitational effects on both air and blood, the matching of ventilation and perfusion (blood flow) in the different regions of the lung is heterogeneous (i.e., V/Q mismatch). From rest to maximal exercise, there is a drastic increase in alveolar ventilation (15–20x), while cardiac output increases a modest 5–7x, which will lead to a larger V/Q mismatch. However, because both ventilation and cardiac output increase during exercise, the lung has a higher V/Q leading to higher PAO2 and PaO2. Thus, the contribution of V/Q to A-aDO2 is similar during exercise to rest; however, in some subjects it can contribute to the majority of the A-aDO2 during maximal exercise [36]. The degree of V/Q mismatch is not different across VO2max [37]. A sex differences does not exist in the degree of V/Q mismatch during exercise [37].
Shunts
Pulmonary shunts are pathways in which blood bypasses the gas exchange portions of the lung. Extrapulmonary (e.g., Thebesian vein and bronchial circulation), intracardiac (patent foramen ovale), and/or intrapulmonary shunts occur at rest and during exercise. The prevalence of intracardiac shunts specifically patent foramen ovale is ~30 % [38] and the prevalence of intrapulmonary shunts is ~90 % [33] in healthy humans. Shunts can lead to impairment of pulmonary gas exchange because the deoxygenated blood is mixed with the oxygenated blood from the lungs returning to the arterial circulation. Approximately 1 % of the total cardiac output flows through shunts [23] and therefore bypasses pulmonary gas exchange. At rest, a portion of cardiac output flows through extrapulmonary and intracardiac shunts [39], which contributes to the resting A-aDO2. During exercise, a portion of cardiac output flows through intrapulmonary shunts [33, 40] and to a lesser degree intracardiac shunts [39]. The increased cardiac output flowing through shunts during exercise contributes to the widening of the A-aDO2. Therefore, shunts can impair resting a nd exercising pulmonary gas exchange, but there is no sex difference in the opening of the shunts during exercise [33].
Diffusion Limitation
Diffusion limitations can also lead to widening of the A-aDO2 during exercise. The contribution of diffusion limitation to the widening of A-aDO2 is similar to V/Q mismatch [23]. A diffusion limitation is any impairment in diffusion of oxygen from the atmosphere to the hemoglobin in the pulmonary capillaries. Diffusion of oxygen is reliant on the rate of equilibrium of the mixed venous blood with the PAO2 (lung diffusion capacity) and the mean transit time of red blood cells through the lung.
Lung diffusion capacity (D LCO) is the capability for the atmospheric oxygen to diffuse across the alveolar-capillary membrane, diffuse across the blood, and bind with the hemoglobin in the pulmonary capillary. Historically, diffusion capacity has been characterized as resistances and simplified in the following equation:
where 1/D M is the resistance to diffusion by the alveolar-capillary membrane and 1/θ*V C is the resistance of uptake of oxygen to the hemoglobin (θ) in the pulmonary blood volume (V C) [41]. Women have smaller lungs, less alveoli (and therefore lung surface area), and lower resting D LCO compared to height-matched men [16, 17]. The lower lung diffusion capacity results from a lower pulmonary blood volume. During incremental exercise, there is a proportional increase in D LCO due to increases in pulmonary blood volume. At rest, pulmonary blood volume is ~70 mL and during exercise it can increase to 150–200 mL because of pulmonary capillary recruitment and distention. These sex differences in lung diffusion capacity and pulmonary blood volume at rest likely exist during exercise. In fact, the D LCO in men at maximal exercise is almost doubled compared to women (64.7 vs. 37.7 mL/mmHg/min) due to their 40 % higher pulmonary blood volume compared to women [125]. A smaller lung diffusion capacity can decrease the rate of diffusion of oxygen from the atmosphere to the pulmonary capillaries impairing pulmonary gas exchange.
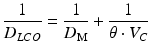
The pulmonary blood volume associated with D LCO is also very important for transit time of the pulmonary red blood cells. Mean red blood cell transit times are calculated by dividing pulmonary blood volume by cardiac output. At rest, the mean transit time is ~0.8 s which is usually adequate time for equilibration. If pulmonary blood volume did not increase during heavy and maximal exercise, pulmonary red blood cell transit times would fall below 0.25 s leading to diffusion limitation and widening of the A-aDO2. The 2–3x increase in pulmonary blood volume is extremely important to allow adequate time for the red blood cell to become oxygenated in the pulmonary capillary during exercise. Mean red blood cell transit time decreases during exercise, but remains above ~0.25 s to allow for complete equilibration. However, in endurance-trained men, cardiac output may increase up to ~30 L/min, while pulmonary blood volume is similar to an untrained individual (~150–200 mL), which can lead to insufficient time for diffusion equilibration and widening of the A-aDO2. The smaller pulmonary blood volume of women during maximal exercise will lead to a lower mean pulmonary red blood cell transit time compared to men. It is quite possible, especially in endurance-trained women, the mean pulmonary red blood cell transit time may fall <0.25 s, which would not allow for complete diffusion equilibrium. The incomplete diffusion equilibrium will lead to pulmonary gas impairments and contribute to the higher prevalence of EIAH in women .
Inadequate Hyperventilation
Inadequate hyperventilation during exercise does not increase the widening of the A-aDO2, but still contributes to the onset of EIAH. Inadequate hyperventilation is present when the PaCO2 is >~38 mmHg during heavy exercise [23]. Inadequate ventilation is due to altered chemosensitivity [43] and mechanical constraints [7]. Specifically, resting hypercapnic and hypoxic chemosensitivity is lower in men who experience EIAH at maximal exercise compared to men without EIAH [43]. Chemosensitivity is not different between men and women [44]. This suggests that the development of EIAH is at least partially due to decreased chemosensitivity in men and women leading to an inadequate hyperventilatory response.
Mechanical constraints of the airways also can limit the hyperventilatory response during exercise. Expiratory flow limitation (EFL ) (explained below) can limit ventilation during heavy exercise. This was demonstrated by using a heliox inspirate (increases the maximum flow -volume loop by ~20–35 %) to reduce EFL during exercise. Without EFL (via the heliox inspirate), alveolar ventilation, tidal volume, and breathing frequency were all higher compared to exercising with EFL [7, 45]. However, breathing the heliox inspirate during heavy to maximal exercise only prevented 30–40 % of EIAH, suggesting that mechanical constraints do not fully explain the development of EIAH [23]. In fact, endurance-trained men who inadequately ventilate during maximal exercise would need to further increase their ventilation ~50 L/min to fully prevent the development of EIAH. However, endurance-trained men use ~85 % of their maximum ventilatory capacity so increasing ventilation ~50 L/min is not possible [23]. Due to morphological differences, women have a higher prevalence of expiratory flow limitation and higher severity of EFL at similar ventilations compared to men [35]. With more of their tidal volume expiratory flow limited, ventilation during exercise is likely to be limited to a greater extent in women compared to men. Additionally, endurance-trained women exhibit more EFL during exercise compared to non-endurance-trained women, suggesting that endurance-trained women are more likely to develop EIAH [7]. The higher prevalence and severity of EFL in women contributes to the inadequate hyperventilation and the increased development of EIAH .
Ventilation
The normal ventilatory response to exercise includes increasing minute ventilation (V E) , which is accomplished by increases in both tidal volume (V T) and breathing frequency (f b). At exercise less than 50 % of vital capacity (VC), V T increases with minimal increases in f b. The greater tidal-volume loop causes decreases in both inspiratory and expiratory reserve volumes (IRV, ERV, respectively), but still is capable of maintaining pulmonary gas exchange and adequate ventilation within the maximum flow-volume loop (MFVL ) [46]. Figure 1.3 shows an MFVL as well as end-expiratory lung volume (EELV ) , end-inspiratory lung volume (EILV ) , inspiratory reserve volume, and expiratory reserve volume [47]. Increases in V T occur to maintain the greatest compliance with the least resistive work of breathing (WOB) in the pressure–volume relationship [48] (see section “Work of Breathing”).
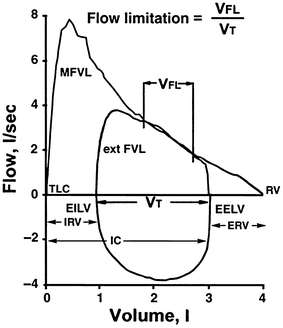
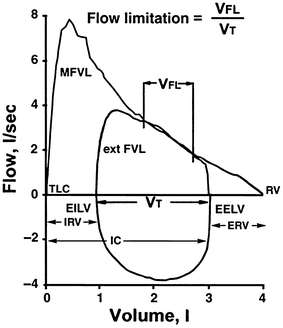
Fig. 1.3
Maximal flow-volume loop and lung volumes . MFVL maximal flow-volume loop, TLC total lung capacity, EILV end-inspiratory lung volume, IRV inspiratory reserve volume, IC inspiratory capacity, V T tidal volume, EELV end-expiratory lung volume, ERV expiratory reserve volume, RV residual volume, ext FVL exercise flow-volume loop, V FL volume expiratory flow limited
The greater V T is accomplished by decreasing EELV below functional residual capacity (FRC) by recruitment of the expiratory muscles. The activity of the expiratory muscles in the reduction of EELV enables greater expiratory flow rates, lowers intra-abdominal pressure, and enables the diaphragm to function at optimal length for generating force [49]. Lastly, the expiratory muscles that allow lung volume to decrease below FRC will cause subsequent decreases in inspiratory muscle work by allowing for the storage of elastic energy in the chest and abdominal walls during expiration. This energy can be used for the following inspiration and reduce the total WOB [50, 51]. Additional increases in EILV also maximize the V T.
During exercise where V E exceeds 50–60 % of VC, further increases in V E are met solely by increases in f b [46]. This is accomplished by decreasing inspiratory and expiratory time (T I and T E, respectively) [52]. There is a greater T E relative to T I relative to total breath time (T TOT), with a T I/T TOT as high as 0.55 during maximal exercise [53]. Regulation of airway caliber also helps achieve high ventilatory rates by decreasing airway resistance during heavy exercise. As inspiratory and expiratory flow increase, dilation and stiffening of intrathoracic and extrathoracic airways occur to reduce turbulent airflow [54, 55]. The pulmonary system is extraordinarily built for maintaining V E, and the increases in V T and f B throughout exercise maximize alveolar gas exchange and minimize WOB. The ventilatory response to increasing exercise is displayed in Fig. 1.4 , adapted from Sheel and Romer [52].
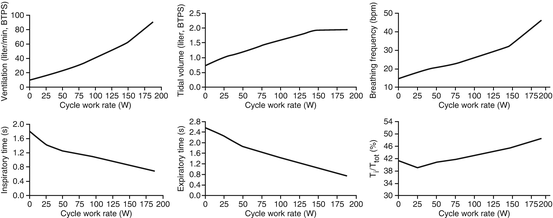
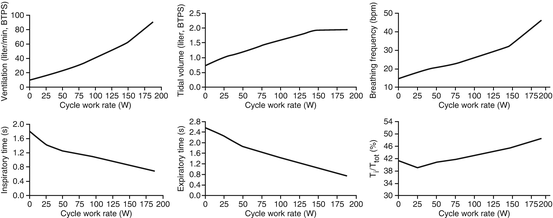
Fig. 1.4
The ventilatory response to incremental exercise during cycling in one healthy subject. There is an initial rise in tidal volume (V T), followed by an increase in breathing frequency when V T plateaus
The literature investigating the breathing mechanics and control of ventilation in men and women at rest and during exercise is contradictory and inconclusive. Current research suggests that exercise values for V E, V T, and f b are similar between men and women, and the plateau in V T is the same in men and women when controlling for FVC [7]. Habedank et al. [56] and Blackie et al. [57] each reported no difference between breathing reserves in men and women, although their reported absolute values were about 20 % different (~61 % reserve and ~41 % reserve, respectively). This discrepancy is more likely due to the mode of exercise utilized in the study design (cycling versus treadmill running) rather than differences in breathing pattern during exercise [58].
The control of V E during exercise is multifaceted. Exercise ventilation during submaximal or steady-state exercise increases in three distinct phases. The first phase is an immediate increase in ventilation upon the onset of exercise, while the second is a further slow, gradual increase between 5 s and 2–3 min. The third is a stable phase where ventilation is sustained for several minutes, but can drift during prolonged time of exercise. Control of ventilation has primarily been attributed to the mechanisms that contribute and regulate exercise hyperpnea to prevent hypercapnia and hypoxemia [46]. These include (1) feedforward influences including central command and CO2 flow, (2) feedback from the exercising limbs, and (3) contribution from carotid chemoreceptors. Feedforward deals with a sensor (e.g., respiratory sensors: chemoreceptors and sensory receptors) transmitting information to the central nervous system, where it integrates the information and further increases or reinitiates signals to the effectors (e.g., the respiratory muscles). Feedback occurs when the sensor transmits information, and when the information is received, there will be a subsequent decrease or increase in drive back to the effectors that will attempt to return the body back to homeostasis. Due to the complexity of control of breathing during exercise, we partitioned the control of breathing into two categories (feedforward and feedback) as has been previously done by Sheel and Romer [52].
Central command (neural signals that originate from the hypothalamus) has a recognized and definite contribution to regulation of exercise hyperpnea, although the magnitude of the input is uncertain. Eldridge and colleagues [59, 60] conducted several studies to understand the role of central command in the control of breathing using an anaesthetized brain model and anaesthetized decorticate cats. This animal model allowed their research team to investigate locomotor, respiratory, and cardiovascular responses when feedback mechanisms were eliminated. The findings concluded respiratory responses began before peripheral feedback mechanisms, indicating feedforward systems contribute to control of breathing that originates in the central nervous system. Carbon dioxide increases at the onset of exercise, and V E closely tracks VCO2 to prevent CO2 and H+ accumulation in arterial blood. Although this is not a causal relationship between VCO2 and ventilation [61], there has been strong evidence from research investigating ventilatory–metabolic coupling via this feedforward mechanism [62–64]. Conflicting research exists, particularly in animal studies that show CO2 flow is minimally altered with exercise hyperpnea [65–67]. Still no known CO2 receptors have been identified so the feedforward mechanism for CO2 flow is based primarily on strong correlative data.
Feedback mechanisms come from (1) the limb locomotor muscles and (2) the carotid chemoreceptors. In skeletal muscles, thinly myelinated muscle afferents (group III and group IV) are present that sense mechanical and chemical changes. During exercise, these afferents are stimulated and send feedback to the medulla to increase V E. Both human and animal studies have used electrical stimulation of these muscle afferents and reported an increase in V E. Still, it remains unclear to what extent these afferents contribute to the exercise hyperpnea. The carotid bodies also provide feedback because they are sensitive to hypercapnia, hypoxemia, hyperkalemia, and acidosis. Studies support the ventilatory response to hypoxia and hypercapnia is greater during exercise than at rest ([68, 69], respectively). However, the carotid bodies may play a larger role during hyperventilation during heavy exercise [70, 71]. For a comprehensive reviw on the control of breathing, refer to Forster and colleagues [123]
Published literature investigating sex differences in the control of breathing yields conflicting and inconclusive results, although the literature is scarce. These existing studies report either an increased V E during exercise while subjects are in the luteal phase compared to the follicular phase [5, 72, 126] or no change between phases [6, 73–75]. Additional studies have reported minimal improvements in VO2max [9] depending on menstrual phase, while others have shown no difference [2, 76, 77]. Jurkowski and colleagues [72] reported a significant improvement in cycling time to exhaustion at 90 % peak power during the luteal phase and conclude the increased time to exhaustion was due to 30 % lower lactate accumulation at end-exercise. Most studies show dissimilar results and show no performance improvements across the menstrual cycle [78, 79].
Expiratory Flow Limitation
Expiratory flow limitation (EFL) is defined a s the point when the tidal-volume loop intersects with the MFVL, as displayed in Fig. 1.5 . Regulation of EELV and EILV, as described earlier, contributes to the ventilatory constraint experienced during exercise [48]. As EELV decreases below FRC, expiratory reserve volume (ERV) is reduced. As the exercising tidal-volume loop encroaches upon the maximal expiratory flow portion of the flow-volume loop, EELV will begin to rise to prevent EFL [80]. This causes EILV to increase and can approach total lung capacity (TLC) [45]. Johnson et al. [47] termed this dynamic hyperinflation , which can cause decreases in elastic muscle strength and inspiratory muscle endurance, and increased work of breathing and oxygen cost of breathing. Dynamic hyperinflation, due to EFL or impending EFL, reduces inspiratory muscle length and compromises force generation [81]. The decreased force generation of the inspiratory muscles places a greater pressure generation on them to operate at higher lung volumes, increasing the WOB.
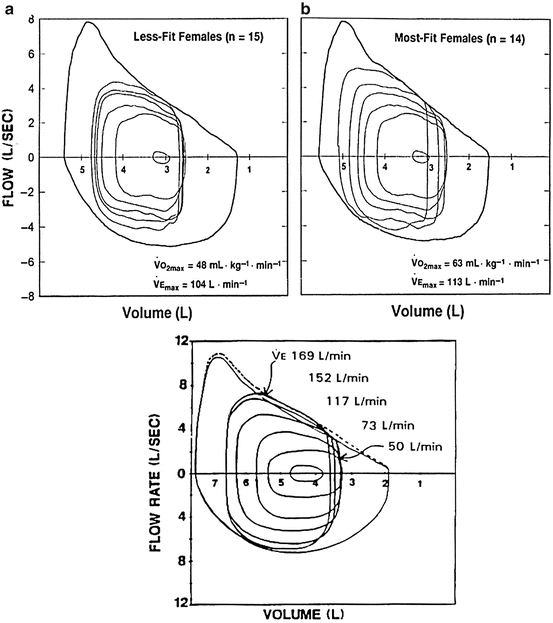
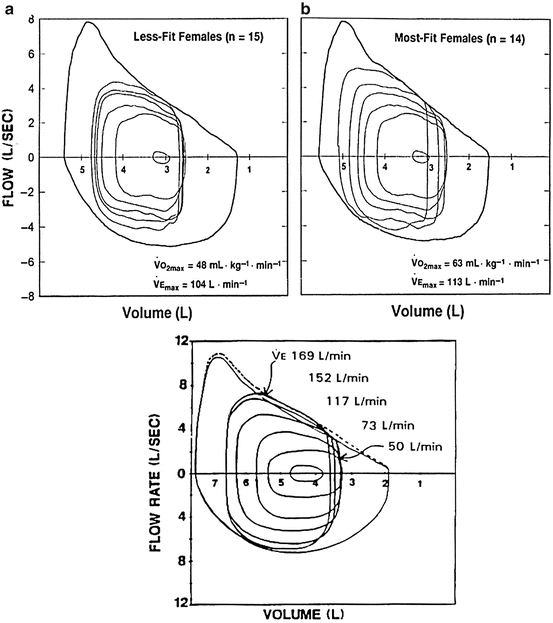
Fig. 1.5
Top represents mean flow-volume loops in young adult women while figure on the bottom represents young adult men: (a) is less-fit women while (b) is highly fit women at rest, then during light (55% VO2max), moderate (74% VO2max), and heavy (90% VO2max), all the way to near maximal (96% VO2max), plotted relative to the group mean maximal flow-volume loop; V Emax, maximal ventilation. Flow limitation occurs when the tidal-volume flow loop intersects with the maximal expiratory flow-volume loop
Smaller airway diameters in women compared to men make women more prone to show EFL during exercise. Women have greater mechanical constraints than men due to a smaller pulmonary envelope compared to that of men [35]. The increased ventilatory drive coupled with the decreased pulmonary envelope contributes to EFL during exercise [7], and women may therefore experience EFL at a lower minute ventilation and oxygen consumption (VO2) than men. Indeed, women experience more EFL at a lower V E and VO2 as well as exhibit more dynamic hyperinflation compared to their male counterparts [35]. As a consequence of the EFL for a given VO2, a woman is more vulnerable to higher levels of WOB that occurs at high exercise intensities and ultimately to other adverse outcomes including increased perception of labored breathing (dyspnea), respiratory muscle fatigue, and gas exchange impairments.
Dyspnea (i.e., the perceived exertion of breathing) increases with exercise, and often limits exercise tolerance via labored breathing and discomfort from both the respiratory system and locomotor muscles. EFL has been reported to be a strong predictor of dyspnea during exercise [82], and imposed EFL in healthy adults causes substantial dyspnea [83]. The perception of discomfort in breathing is associated with increases in inspiratory muscle work from EFL and may limit exercise tolerance.
< div class='tao-gold-member'>
Only gold members can continue reading. Log In or Register a > to continue
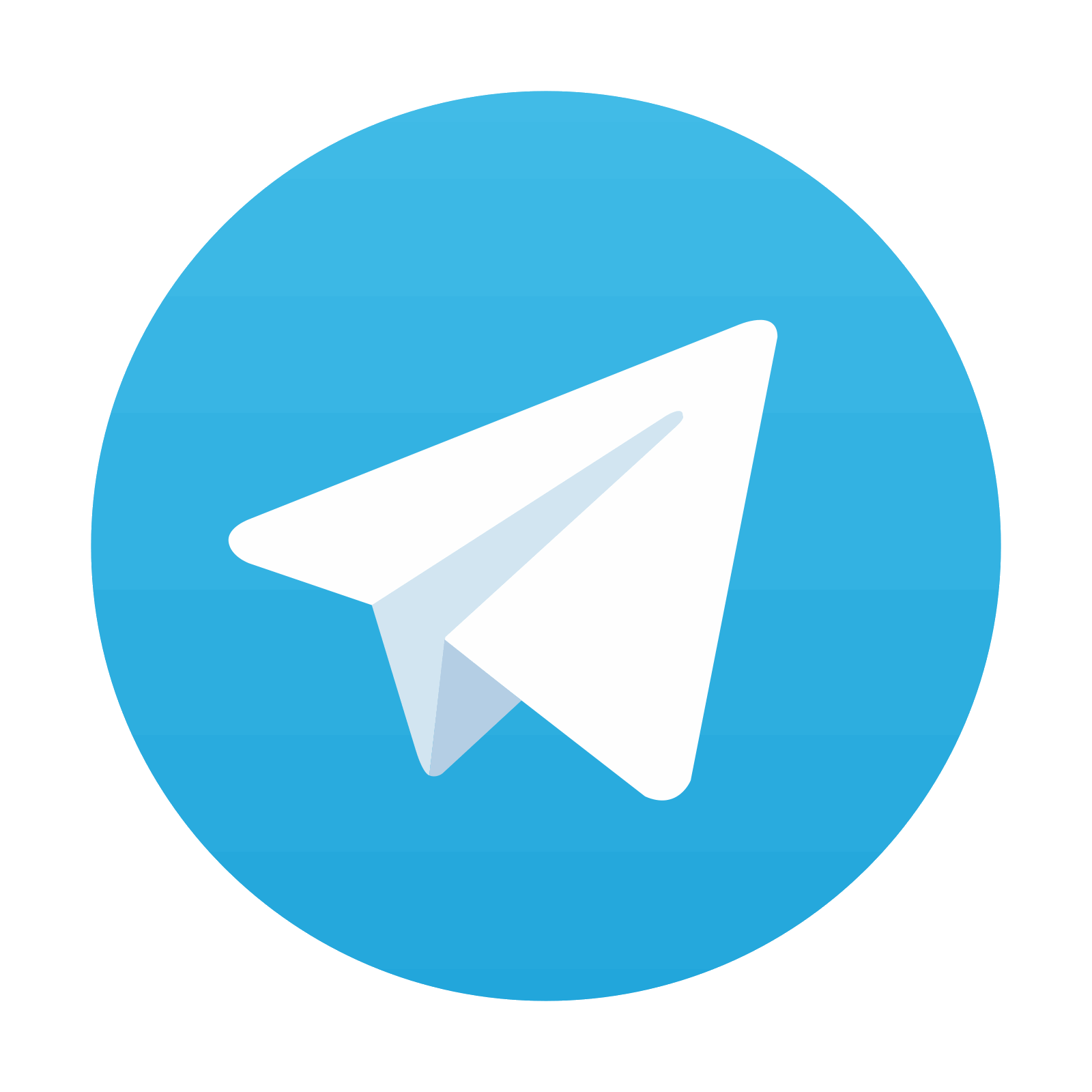
Stay updated, free articles. Join our Telegram channel
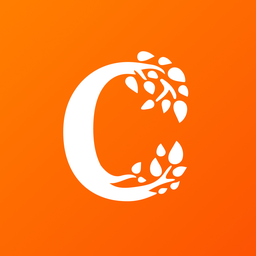
Full access? Get Clinical Tree
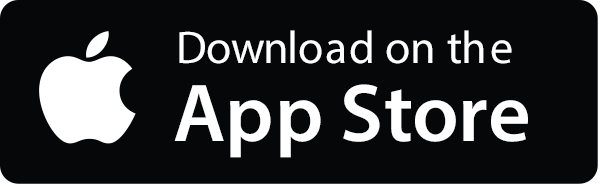
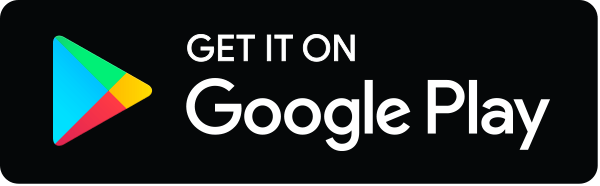