Baseline
During cancer therapy
Following completion of cancer therapy
Heart Failure Association—European Society of Cardiology (2011) [40]
Careful cardiovascular work-up with attention to comorbidities esp. CAD and hypertension
Regular cardiovascular evaluation
Follow-up cardiac surveillance should be considered especially in those patients receiving high doses of anthracyclines
European Society of Medical Oncology (ESMO, 2012) [38]
Baseline cardiovascular evaluation (risk factors and comorbidities)
Baseline 12-lead ECG and echocardiogram or MUGA
Baseline biomarker (troponin, BNP) treatment of preexisting cardiopathies
Patients receiving anthracyclines +/- trastuzumab should have serial monitoring of cardiac function at 3, 6, and 9 months during treatment and then 12 and 18 months after initiation of treatment
Biomarkers with each cycle
Monitoring following treatment as clinically indicated
Increased vigilance for patients >60 years old
Assessment of cardiac function recommended 4 and 10 years after anthracyclines in patients treated <15 years old and those >15 years old with a cumulative dose of doxorubicin >240 mg/m2 or epirubicin >360 mg/m2
European Association of Cardiovascular Imaging and American Society of Echocardiography (2014) [41]
Baseline cardiac assessment especially for those >65 years old and at high risk of CTRCD, LV dysfunction, or high doses of anthracyclines (>350 mg/m2)
History, physical examination, ECG, echocardiogram, and baseline global longitudinal strain (GLS), and troponin I desirable
Anthracyclines: troponin each cycle; if positive: cardiology consult. If dose >240 mg/m2 or equivalent: recommended LVEF, GLS, and troponin prior to each additional 50 mg/m2.
Trastuzumab: LVEF ,GLS, and troponin every 3 months during therapy and 6 months after treatment completed
Yearly cardiovascular assessment by a health-care provider particularly in patients not followed closely during cancer treatment imaging at the discretion of the health-care provider
In 2012, the European Society of Medical Oncology (ESMO) published criteria-based recommendations of optimal screening and monitoring of cardiac function during cancer therapy based on a review of current evidence. Similar to the ESC, they recommended baseline assessment of cardiovascular risk factors and comorbidities. In addition, ESMO recommended patients receiving potentially cardiotoxic therapy have a baseline 12 lead ECG, assessment of LVEF with echocardiogram or MUGA , and measurement of biomarkers including troponin and BNP. Cardiac monitoring during cancer therapy focused on patients receiving anthracyclines +/- trastuzumab. For patients receiving anthracyclines, measurement of LVEF was recommended (in the absence of baseline troponin) every 3 months for 1 year followed by yearly assessments. In patients with normal troponin levels (during anthracycline chemotherapy ), echocardiogram was recommended at 12 months and then yearly thereafter. Patients receiving trastuzumab should have LVEF assessed every 3 months during therapy and then at 12 and 18 months following initiation of treatment. Following completion of cancer therapy, cardiac assessment was recommended at 4 and 10 years in patients with higher exposure to anthracyclines (>240 mg/m2 Adriamycin ) with increased vigilance in those patients over 60 years of age. ESMO also provided stop/go rules for individuals receiving trastuzumab therapy [38]. Both ESC and ESMO reinforced that newer targeted molecular agents are associated with adverse cardiovascular events and that the early detection of cardiotoxicity needs to be an integral part of the treatment and follow-up of cancer patients [42].
In 2014, the American Society of Echocardiography and the European Association of Cardiovascular Imaging published an expert consensus on surveillance strategies for adult patients during and after cancer therapy [41] (see Table 3.1). This group recommended that echocardiography remain the cornerstone in the cardiac imaging evaluation of patients in preparation for, during, and after cancer therapy, because of its widespread availability, easy repeatability, versatility, lack of radiation exposure, and safety, particularly in patients with concomitant renal disease. Similar to ESMO, they suggested that an integrated approach combining echocardiography and biomarkers may be of utility and provide incremental value in predicting subsequent cancer therapy-related cardiac dysfunction. After the completion of therapy and particularly among patients who were not followed using a strategy aimed at early detection of subclinical LV dysfunction, the committee suggested a yearly clinical cardiovascular assessment by a health-care provider, looking for early signs and symptoms of cardiovascular disease, with further cardiac imaging ordered at the discretion of the provider. The committee highlighted that their recommendations represent a consensus of current clinical practice performed at their own respective academic institutions. Similar to other consensus statements, the group recognized the limited scientific data available and the lack of class A evidence (i.e., derived from randomized clinical trials) supporting their algorithms.
While these consensus documents provide guidance to clinicians, the impact of cardiac monitoring during and following completion of cancer therapy, on cardiovascular outcomes in cancer patients being managed in the “real-world” clinical setting, is uncertain. In addition, there is limited data in the literature on the adherence to cardiac monitoring recommendations in clinical practice. In a recent US study, 78.8 % of postmenopausal women (66 years and older) with early-stage HER2-positive breast cancer receiving trastuzumab had a baseline cardiac evaluation, while only 42.6 % had subsequent monitoring (one cardiac evaluation at least every 4 months during trastuzumab therapy ). Recent year of cancer diagnosis and treatment with an anthracycline regimen was associated with higher rates of adequate cardiac monitoring [43].
Cardiovascular surveillance strategies for cancer patients have been largely limited to anthracyclines and anti-HER2 drugs such as trastuzumab. Several new classes of promising anticancer therapies have been introduced into clinical practice, several of which have unique cardiovascular toxicities (see Table 3.2). Tyrosine kinase inhibitors have demonstrated efficacy in a number of solid malignancies (renal cell carcinoma (sunitinib), hepatocellular (sorafenib), and head and neck (cetuximab)), but are associated with development of de novo or worsening hypertension. While strategies on the management and monitoring of cancer patients who develop hypertension while receiving these novel targeted drugs are emerging, there are currently no consensus or evidence-based documents to guide therapy. In the interim, patients should be managed based on current hypertension guidelines produced by organizations such as the American Society of Hypertension (www.ash-us.org), Hypertension Canada (http://guidelines.hypertension.ca), and the European Society of Hypertension (www.eshonline.org). A number of emerging cancer therapies are associated with less common cardiovascular toxicities including QTc prolongation, arrhythmias , cardiac ischemia, and thromboembolic events (see Table 3.2). Monitoring strategies for these cancer therapies should follow current evidence-based international guidelines (American (www.heart.org), Canadian (www.ccs.ca), European (www.escardio.org), UK (www.bhf.org.uk, heartuk.org.uk)).
Table 3.2
Cardiotoxicity and cancer therapies
Anticancer therapy | Signs and symptoms of toxicity |
---|---|
Anthracyclines (Doxorubicin, daunorubicin, idarubicin, epirubicin, mitoxantrone) | (1) Acute toxicity: <1 %, reversible, shortly after infusion Toxicities include arrhythmias, QT prolongation +/-HF (2) Early-onset chronic progressive: 1.6–2.1 %, during treatment and up to 1 year post, not reversible, clinically resembles myocarditis, accompanying diastolic dysfunction (3) Late-onset chronic progressive: 1.6–5 %, >1 year from treatment, not reversible, clinical decompensation usually preceded by occult LVD Symptoms of mitoxantrone-induced HF are often less severe and more responsive to medical management |
Cyclophosphamide | (1) Arrhythmias (2) Nonspecific ST-T abnormalities (3) Pericardial effusion (4) Hemorrhagic myopericarditis (5) Symptomatic HF (7–28 %) Occurs within 1–14 days of dose administration and often lasts for a few days. Toxicity may resolve completely or have long-lasting consequences |
Ifosfamide | (1) Arrhythmias (2) Nonspecific ST-T changes on ECG (3) HF 17 % Acute HF typically presents within 6–23 days of first ifosfamide dose |
Cisplatin | (1) Chest pain (2) Arrhythmias (3) ST-T changes on ECG (4) ACS (5) Thromboembolism (8.5 %) |
Antimetabolites | |
Fluorouracil (5-FU) | (1) Chest pain or ACS in 3–7.6 % (2) Atrial fibrillation (3) HF (4) Sudden cardiac death (rare) Occurs during or shortly after starting treatment. Symptoms last up to 48 h, but generally resolve. ECG changes present in up to 68 % of patients treated with continuous infusion. Elevated cardiac biomarkers in up to 43 % |
Capecitabine | (1) Chest pain or ACS (3–9 %) with transient ST elevations on ECG Symptoms occurs 3 h–4 days after initiating therapy Cardiac biomarkers generally remain normal |
Microtubule-targeting agents | |
Paclitaxel | (1) Myocardial ischemia (1–5 %) (2) MI (0.5 %) (3) Arrhythmias and heart block Cardiac complications occur in up to 29 % with most being asymptomatic bradyarrhythmias. Occur during and up to 14 days after paclitaxel administration. Symptoms generally resolve with stopping therapy |
Docetaxel | (1) HF 2.3–8 % (2) Myocardial ischemia (1.7 %) |
Tyrosine kinase inhibitors | |
Sunitinib | (1) Hypertension (47 %) (2) Asymptomatic decline in LVEF (10–21 %) (3) Symptomatic HF in up to 15 % Variable time to presentation (days–months) |
Sorafenib | (1) MI (2.7–3 %) (2) Hypertension (17–43 %) (3) HF/LV dysfunction Less cardiac dysfunction than sunitinib |
Axitinib | (1) Hypertension |
Regorafenib | (1) Hypertension |
Vandetanib | (1) Torsades de pointes |
Imatinib | (1) LVEF reduction (0.5–1.7 %) |
Dasatinib | (1) HF/LV dysfunction |
Lapatinib | (1) LV dysfunction (1.6–2.2 %) (2) Symptomatic HF (0.2–1.4 %) (3) QTc prolongation Relatively low incidence of adverse cardiac events |
Monoclonal antibodies | |
Trastuzumab | (1) HF/LV dysfunction with variable rates based on definitions from clinical trials 2–7 % as monotherapy, 2–13 % with paclitaxel Up to 27 % with anthracyclines |
Bevacizumab | (1) HTN (2) HF (0.8–22 %) (3) MI/angina (1.5 %) (4) ATE during treatment (median, 3 months) |
Radiation therapy | (1) CAD (2) Valvular disease (3) Pericardial disease (4) Restrictive cardiomyopathy (5) Conduction system disease |
In recognition of the increasing need for guidance, the ASCO Survivorship Guidelines Advisory Group has recently published a guideline to improve the quality of care of cancer survivors by identifying and providing guidance on prevention and monitoring of cardiac dysfunction resulting from cancer therapy; and the Canadian Cardiovascular Society has recently completed a guideline on evaluation of patients at risk for cardiovascular complications of cancer therapy [44, 45].
Defining Cardiotoxicity
Anthracycline-related symptomatic HF was first described in the 1970s [15]. In the 1970s and 1980s, multigated acquisition (MUGA) scanning, also known as resting radionuclide angiocardiography, emerged as the modality of choice for monitoring LV function in adult and pediatric patients treated with anthracyclines [46–49]. Initial guidelines were proposed by Alexander et al. grading heart failure (HF) as mild, moderate, or severe, on the basis of a progressive fall in LVEF using serial MUGA imaging [46]. These guidelines were implemented in a large single-center study over 7 years, and the use of these guidelines in conjunction with MUGA for monitoring LVEF was demonstrated to be associated with a low incidence, benign course, and reversible degree of anthracycline-related HF [49]. Accordingly, assessment of LV systolic function by resting LVEF has become routine in current practice for cardiovascular evaluation in cancer patients treated with anticancer therapy [46]. Among non-cancer populations, LVEF has been repeatedly shown to be an important and independent prognostic indicator and is used frequently in clinical decision-making [31, 50, 51]. In a cancer population, the prognosis of developing LV systolic dysfunction is presumably worse compared to the general population, given the continuous nature of the myocardial insult from cytotoxic therapy.
However, there are limitations with the current paradigm that uses resting LVEF for surveillance of changes in cardiac and cardiovascular function during and after potentially cardiotoxic anticancer therapy. First, resting LVEF provides a snapshot of cardiac performance under optimal circumstances and may not demonstrate subclinical loss of cardiac reserve from early myocyte damage [52]. Second, resting LVEF principally assesses load-dependent changes in LV cavity size. Changes in loading conditions during chemotherapy are common, and, as a result, LVEF may not reflect actual myocardial systolic function [53]. In fact, LVEF may overestimate actual LV health when intrinsic mechanisms are initially compensatory to maintain cardiac output in the face of acute myocardial injury [54]. When such compensation ultimately falters, a drop in LVEF is finally observed perhaps too late to avert irreversible cardiomyopathy and attenuate cardiac events [55, 56]. Third, cancer therapy-induced damage often extends beyond the heart and may occur in conjunction with (mal) adaptation in other organ components, which are not evaluated by resting LVEF. Many anticancer therapies cause unique and varying degrees of injury to the cardiovascular system (i.e., pulmonary-vascular/blood-skeletal muscle axis) [57]. For example, radiation and certain forms of systemic therapy (e.g., chemotherapy , molecularly targeted therapies) can cause pulmonary dysfunction, anemia, endothelial dysfunction, and pulmonary/systemic arterial stiffness as well as skeletal muscle dysfunction [11, 12, 58–60]. These direct insults occur in conjunction with “indirect” lifestyle perturbations (e.g., physical inactivity) that synergistically cause marked impairments in cardiovascular reserve capacity (CVRC) [61].
Moreover, accurate assessment of the frequency and magnitude of cardiotoxicity is challenging given differences in LVEF cut-points, monitoring frequency, and measurement modalities used in clinical trials that limit direct comparisons. There are currently no consensus criteria for cardiotoxicity [38, 41, 62]; trial-based cardiac safety end points have been heterogeneously defined as either (1) an absolute reduction of LVEF >10 % or >15 %, (2) an LVEF reduction >10 % or >15 % to a threshold of <55 % or <50 %, or (3) any LVEF decline to < 50 % [63]. Echocardiography , MUGA , or both have been used most frequently for cardiac assessments in clinical trials and practice, although LVEF measurements by these modalities are not interchangeable [64]. The validity of assumptions common to the spectrum of cardiotoxicity criteria, specifically whether an LVEF decline is always attributable to treatment-related toxicity and whether the absence of an LVEF decline can be interpreted as absence of cardiotoxicity, is also debatable [65]. Data addressing the utility of systematic follow-up LVEF assessments in adult cancer survivors with a history of cytotoxic therapy are also lacking [39]. For certain cardiotoxic agents, such as trastuzumab, serial LVEF measurements are Food and Drug Administration (FDA) -mandated in the USA [66], and stopping/holding rules (i.e., LVEF decrease of ≥16 % or 10–15 % below institutional lower limits of normal) that were used in the trastuzumab clinical trials [67–69] during treatment have translated to clinical monitoring practices. The most recent definition of cardiotoxicity proposed by Expert Consensus for Multimodality Imaging Evaluation of Adult Patients during and after Cancer Therapy in 2014 [41] is a decrease in the LVEF of >10 percentage points, to a value <53 %. Moving forward it will be imperative that a common definition of cardiotoxicity be adopted in order to gain a better understanding of cardiac dysfunction in cancer patients exposed to anticancer agents.
Available Modalities for Screening and Monitoring for Cardiotoxicity
Conventional Imaging Modalities
In current practice, multigated acquisition (MUGA) scanning and two-dimensional echocardiography (2DE ) are most frequently used to evaluate resting LVEF .
MUGA uses 99mTc-labeled erythrocytes to visualize the cardiac blood pool with gated acquisition using a gamma camera. A series of planar images at each stage of the cardiac cycle are acquired for the quantification of left ventricular volumes and LVEF (Fig. 3.1). Following the findings of Schwartz et al. [49], MUGA soon became popular as the technique of choice for monitoring cardiotoxicity, and detection of an asymptomatic decline in LVEF by MUGA was preferred over surveillance for the development of CHF symptoms. Serial MUGA imaging also allowed the administration of high cumulative doses of anthracyclines and the use of anthracyclines when the baseline LVEF was abnormal. MUGA was also demonstrated to have high reproducibility and low variability, making it well suited for serial use [48, 70]. On the basis of this body of evidence, MUGA came to be widely used in oncology clinical trials and in clinical practice. This practice still continues within the oncology community, while the cardiology community has largely moved away from MUGA in favor of echocardiography and cardiac magnetic resonance (CMR) imaging for the evaluation of LV systolic function. Cardiologists favor echocardiography and CMR because these modalities provide additional clinically valuable information not provided by MUGA such as assessment of diastolic function, right ventricular size and function, atrial size and function, valvular disease, pericardial disease, intracardiac thrombus, and extra-cardiac pathology [71]. A recently published study of 2203 breast cancer patients from the SEER-Medicare cohort who received adjuvant trastuzumab showed that 42 % were monitored by echocardiogram, 28 % were monitored by MUGA, and 23 % had imaging alternating between the two modalities [72].


Fig. 3.1
Example MUGA images from cancer patients. (a) and (b) are end-diastolic and end-systolic MUGA images, respectively, from a 61-year-old male whose LVEF was analyzed to be abnormal at 47.0 %; (c) and (d) are end-diastolic and end-systolic MUGA images, respectively, from a 61-year-old female whose LVEF was analyzed to be normal at 69.2 %
Advantages of MUGA imaging include the ability to image almost all patients without limitations due to body size or obesity, poor acoustic windows, or the presence of cardiac devices such as pacemakers or defibrillators. MUGA is also widely available [71]. Limitations of MUGA include the potential of lower hematocrit and commonly used drugs such as digoxin, heparin, prazosin, and hydralazine to adversely affect the efficiency of erythrocyte labeling [73]. Medications that decrease erythrocyte labeling will reduce the target-to-background ratio and lead to error. Due to the need for cardiac gating, the accuracy of LVEF assessments is limited by arrhythmias such as atrial fibrillation [73]. MUGA is also associated with an average typical effective ionizing radiation dose of 8 mSv [74, 75]. Thus, in a hypothetical breast cancer patient receiving adjuvant trastuzumab who is recommended to have LVEF assessment before starting treatment, every 3 months during, upon completion of treatment, and every 6 months for at least 2 years following completion of treatment [76], the use of MUGA would result in a cumulative effective dose of 72 mSv. Based on published estimates of the radiation-related secondary cancer risk from technetium-99 m myocardial perfusion studies [77], a hypothetical 50-year-old female who undergoes nine MUGAs would be estimated to have a lifetime risk of 0.64 % for a radiation-related secondary cancer. This is not an insignificant risk when considered in light of the excellent survival rates for patients diagnosed with breast cancer today—a 5-year relative survival rate of 89 % and a 10-year relative survival rate of 82 % [78]. Finally, the high reproducibility of LVEF measurements reported in the 1970s and 1980s may not apply to current gamma cameras. Those early MUGA studies were performed using small-field-of-view, single-headed gamma cameras that allowed optimal positioning of the patient and good separation between the left and the right ventricles. Current gamma cameras are primarily large-field-of-view, dual-headed systems that do not permit this degree of patient positioning [71].
Two-dimensional echocardiography has emerged as the primary cardiac imaging modality in patients who are preparing for, are receiving, or have completed anticancer therapy. Accurate measurement of LVEF by 2DE requires sufficient visualization of the LV endocardial border to enable manual tracing of the end-systolic and end-diastolic volumes from which LVEF is calculated. Visual LVEF assessments by 2DE are also common in echocardiography laboratories in clinical practice [79] and prior studies suggest visual LVEF assessments correlate with quantitative methods [79, 80]. Echocardiography has well-described disadvantages, including reliance on adequate acoustic windows and assumptions of LV geometry (for 2DE). These limitations diminish the reproducibility and accuracy for assessment of LV volumes and LVEF by 2DE and reduce sensitivity among serial measurements for detection of small changes in LV function; on occasion, measurement variability of LVEF by 2DE may be higher than thresholds used to define cardiotoxicity [81]. However, 2DE has many significant advantages as well, including wide availability, portability, ease of use, and safety given its lack of exposure to radiation and to potentially nephrotoxic contrast agents . Finally, 2DE without contrast does not require intravenous access.
Novel Imaging Modalities
Transthoracic Echocardiography
Three-dimensional echocardiography (3DE) preserves many of the advantages of echocardiography while mitigating some of the limitations that exist with 2DE. In adjuvant breast cancer, 3DE was superior to 2DE in terms of accuracy and reproducibility, and had similar accuracy to MUGA [with cardiac magnetic resonance (CMR) imaging as the gold standard] for LVEF in early breast cancer [82]. Similarly, 3DE may provide more reliable detection of LVEF changes, which is clinically important given the threshold magnitude of LVEF decline (10 %) used to adjudicate subclinical cancer therapy-related cardiac dysfunction by existing criteria [62]. In a prospective comparison of 2DE and 3DE in 56 breast cancer patients, Thavendiranathan et al. [81] found that the temporal variability of LVEF by 3DE was 5–6 % compared with 10–13 % by 2DE. In the same study, non-contrast 3DE measurement of LVEF had the best intra- and interobserver as well as test-retest variability, compared with 2DE. Despite its superiority for LVEF assessments, 3DE requires greater expertise and is less available than 2DE, limiting its use in standard monitoring at most centers.
While evaluation of contractile function with echocardiography has traditionally been limited to volume-based assessment of LVEF and assessment of regional wall motion or visual estimation of regional thickening, there has been interest in the past few years in techniques that provide more objective and reproducible measures of contractile function through imaging of myocardial deformation. Deformation imaging, in the broadest sense, allows for direct assessment of myocardial muscle shortening and lengthening throughout the cardiac cycle. There are several indices available to assess myocardial deformation, including strain, strain rate, and torsion. Current echocardiographic assessments of myocardial deformation are based on tissue Doppler imaging (TDI) or speckle-based tracking (STE) , the two-dimensional tracking of unique speckle patterns created by the constructive and destructive interference of ultrasound beams within tissue, which has technical advantages compared to TDI. These speckles are tracked on a frame-by-frame basis and the accuracy of speckle tracking has been validated against sonomicrometry and tagged CMR imaging [83]. Strain reflects the global deformation of ventricular myocardium during the cardiac cycle, is typically measured at peak systole (i.e., at aortic valve closure), and can be determined in the longitudinal (GLS), radial (GRS), and circumferential (GCS) planes [83]. Beyond measuring linear deformations, peak systolic LV torsion by STE can also measure myocardial rotational deformation due to helical orientation of the myocardial fibers, by calculating the maximum instantaneous difference between peak systolic apical rotation relative to basal rotation [84].
These parameters have emerged as more sensitive measures of subclinical LV dysfunction and may be useful approaches for the early detection of cancer therapy-induced cardiotoxicity and for following patients longitudinally [85]. Reduced TDI strain and strain rate revealed impaired myocardial function prior to LVEF decline [86–88] and HF symptoms [89] in patients treated with anthracycline-containing therapy although the predictive value of these parameters was not evaluated. Global longitudinal strain by STE detects subclinical LV systolic dysfunction among cancer patients with preserved LVEF , including early-stage breast cancer patients undergoing anthracycline-trastuzumab therapy [85, 90] and adult survivors of pediatric malignancies treated with anthracyclines and/or chest radiation [91]. In separate small studies, Sawaya et al. [90, 92] found that impaired absolute GLS (>−19 %) at 3 months and Negishi et al. [93] found that relative GLS decline (≥11 %) from baseline to 6 months predicted LVEF decline in breast cancer patients treated with trastuzumab with or without anthracyclines (Fig. 3.2). GLS also appears to provide superior prognostic information to LVEF in non-cancer populations; a recent meta-analysis showed that GLS independently predicted mortality better than LVEF in 5721 patients with diverse cardiac conditions including HF, acute myocardial infarction, valvular heart disease, and cardiac amyloidosis [51]. Impairments in torsion and torsion velocities in the absence of LVEF changes have been observed 1 month after anthracycline therapy in 25 leukemia/lymphoma patients [94] and after 7 years in 35 anthracycline-treated childhood cancer survivors [95]. Finally, three-dimensional (3D) STE is a novel modality that can comprehensively assess LV myocardial mechanics, tracking linear myocardial deformation in multiple dimensions simultaneously as well as torsion and mechanical dyssynchrony , and has demonstrated early promise in this context, with sensitive detection of altered LV mechanics in childhood cancer survivors treated with anthracyclines [96].


Fig. 3.2
Echocardiographic strain imaging for early detection of cardiotoxicity. In many disease states, measurements of global longitudinal strain (GLS) by two-dimensional echocardiographic speckle tracking are more sensitive for detecting impaired myocardial systolic performance than left ventricular ejection fraction (LVEF) and less susceptible to alterations in loading conditions. In toxin-induced myocardial damage, both in the preclinical and clinical setting, reduced strain and strain rate have revealed impaired myocardial function prior to LVEF decline and heart failure symptoms
Despite their potential, echocardiographic myocardial deformation indices have important considerations currently limiting their widespread use in cardio-oncology. The optimal timing for assessments in relation to chemotherapy completion and cutoff values for positive tests remain undetermined. Strain echocardiography is dependent on high-quality images, has variability related to different vendor acquisition and analysis platforms, and has uncertainty regarding the optimal parameter of myocardial deformation and interinstitutional reproducibility [41]. However, longitudinal strain has been demonstrated to be more reproducible [97] and the emerging evidence suggests myocardial deformation indices have a potential role for early detection of therapy-related cardiotoxicity that merits further investigation and validation in large cohort studies.
Cardiac Magnetic Resonance
Cardiac magnetic resonance (CMR) imaging became a routine clinical test only about a decade ago. This was made possible by dramatic advances in technology, which changed this modality from providing mostly static, tomographic images of organ morphology to high-resolution, dynamic images allowing the assessment of cardiac function with excellent resolution and contrast. Moreover, advances in tissue characterization techniques, have made CMR the gold standard for assessment of myocardial viability, fibrosis, infiltrative, and inflammatory diseases [98, 99]. In this section we will discuss established and routinely available CMR techniques for cardiotoxicity screening during cancer treatment, which at present relies primarily on imaging left ventricular systolic function. Furthermore, we will discuss which additional information can be obtained in the same routine CMR exam for monitoring cardio-oncology patients, namely, early detection of myocardial tissue damage, impaired myocardial blood flow, tumor involvement of the heart and pericardium, and thrombus formation. We will also address promising, new technological developments , which are being explored for use in this patient population.
CMR for Evaluation of Ventricular Function and Volumes
CMR is widely accepted in the cardiac imaging community as the gold standard for assessment of cardiac volumes, left ventricular mass, and function [100] and endorsed by the American College of Cardiology/American Heart Association as a method to screen for chemotherapy -related cardiotoxicity [101]. From a conceptual standpoint, the key parameter for being able to detect changes in ventricular function on serial imaging is the inter-study reproducibility of the imaging modality used for LVEF measurements. This is determined by the standard deviation (SD) of the mean difference between repeat imaging studies. With wider margins in variability (usually expressed as ±2 SD), the ability to detect these subclinical, often small, changes in LVEF decreases. As a consequence, patients may develop HF symptoms without detecting a significant reduction in LVEF in serial imaging. Conversely, important treatment may be held due to a “measurement error,” without a true decline in LVEF. Some information of the relative reproducibility of modalities can be gleaned from prior studies where both image acquisitions and LVEF measurements were repeated in the same patients. Grothues et al. studied 60 patients twice with 2D echocardiography and CMR and found superior reproducibility of LVEF measurements with CMR compared with 2D echocardiography with a mean difference and SD of −0.5±1.7 and 0.5±5.6, respectively [102]. Similar direct comparisons of LVEF measurements of MUGA vs. CMR are not available; however, the reported mean differences for repeated MUGA LVEF measurements are 1.8 %, with SD ranging between 4.4 and 6.9 % [103, 104]. To illustrate, a 5 % SD of the mean difference means that in repeat assessments, notably without any true change in LVEF, this imaging modality will produce LVEF results that are within 20 % of each other (i.e., ±2 SD). With that in mind, it may not be surprising that Swain et al. found in their analysis of 630 patients receiving doxorubicin that 66 % of patients who developed HF had no significant LVEF reduction noticed on serial MUGA scans and many patients had similar EF changes without HF occurrence [16]. The authors concluded that LVEF measurements [with MUGA] are not an accurate predictor of HF in patients who receive doxorubicin. The question though is whether the reproducibility of the modality rather than the physiologic concept of LVEF monitoring is flawed, knowing that occurrence of HF symptoms in the context of cardiotoxicity is uniformly associated with a significant drop in LVEF .
Current guideline cutoffs for detecting cardiotoxicity are based on studies using MUGA showing LVEF variability of 5.4 ± 4.4 % in normal patients and 2.1 ± 2.0 % in abnormal patients [47]. Since the variability of CMR is lower, using CMR we may be able to reliably detect smaller changes and diagnose cardiotoxicity earlier, before there is a large (>10 %) drop in LVEF . Therefore, further research is needed on using CMR LVEFs to detect cardiotoxicity, and based on this research, guidelines may need to be refined. One study supporting this thought is by Drafts et al. [105] who found smaller changes in LVEF of <10 % (58 ± 1 % to 53 ± 1 %), whereas other features such as strain, pulse wave velocity, and biomarkers confirmed that these patients did have cardiotoxicity even though they technically did not meet the current guideline cutoffs for change in LVEF.
Although CMR has been used in cardiotoxicity screening with substantial improvements in diagnosis of cardiomyopathy after cancer treatment [106], at present its use in oncology practice is limited. Arguments raised against its use are primarily concerns regarding availability and cost [62]. Concerning the latter, a closer look at 2014 Medicare National Coverage Determinations Manual demonstrates however a de facto lower cost for the pertinent CMR procedure compared to MUGA and echocardiography procedures (CPT 75557 “cardiac magnetic resonance imaging for morphology and function without contrast material” at $ 294.78 vs. CPT 78473 “cardiac blood pool imaging, gated equilibrium wall motion with ejection fraction” at $ 397.32 vs. CPT 93306 “echocardiography, transthoracic” at $ 427.00) [107, 108]. Second, the USA has a high availability of MRI scanners with a total of about 12,000 scanners or 38 MRI scanners per million population, and the basic functionality for LVEF measurements can easily be implemented on a clinical scanner and performed with little additional cost and training.
Thus, the main practical limitations to performing MRI for screening in cancer arise in patients with pathologic claustrophobia, which in most patients is mild and can be overcome with conscious sedation, and with implantable devices such as older non-MRI compatible pacemakers and implantable cardioverter-defibrillators. Some breast tissue expanders have magnetic ports, such as the Contour Profile Tissue Expander (Mentor, Santa Barbara, CA), and are not considered safe for an MRI examination. Using current state-of-the-art CMR technology, LVEF measurements can be accomplished within a 15 min examination, which does not require intravenous access or contrast administration. Off-line image analysis of the 3D data set can be accomplished on commercially available workstations within 5 min (Figs. 3.3 and 3.4). Importantly, there are no limitations to visualizing the heart due to acoustic window (which may be obscured by breast expanders such as saline or silicone implants, bone or lung tissue), and radiation doses typically in the order of 7 mSv for each serial MUGA scan can be avoided in these patients with already high radiation exposure due to the need for cancer staging [109].



Fig. 3.3
Example CMR images demonstrating cardiomyopathy . A 47-year-old patient with breast cancer treated with anthracyclines, demonstrating cardiomyopathy. Panel A shows a diastolic frame and Panel B shows a systolic frame. The LVEF was quantified at 42 %

Fig. 3.4
Example CMR images demonstrating valvular disease. A 61-year-old patient with lymphoma treated with chemotherapy and radiation, demonstrating aortic stenosis (a) and aortic regurgitation (b)
Delayed Enhancement CMR for Myocardial fibrosis
Cardiac fibrosis can occur without a decrease in LVEF [110, 111] and is a highly sensitive marker of structural heart disease. Cardiac fibrosis occurs in two forms—reactive and replacement. In reactive fibrosis, collagen accumulates diffusely in perivascular and interstitial tissues without cardiomyocyte loss, while replacement fibrosis involves loss of cardiomyocytes. Cardiac fibrosis plays an important role in the development and progression of systolic and diastolic heart failure [112, 113]. Increasing cardiac fibrosis results in progressive deterioration of cardiac function, with more extensive cardiac fibrosis identified in patients with advanced heart failure, regardless of the etiology of cardiomyopathy [114, 115]. The presence and extent of cardiac fibrosis has been associated with heart failure [114] and death [115, 116], even in subjects without known cardiac disease [110, 117, 119]. In recent years, cardiac fibrosis has been demonstrated to have an adverse prognostic impact in various forms of heart disease such as ischemic heart disease [120], nonischemic dilated cardiomyopathy [121], hypertrophic cardiomyopathy [122], cardiac sarcoidosis [123], cardiac amyloidosis [124], myocarditis [125], and aortic stenosis [126]. Thus, cardiac fibrosis is not only a highly sensitive but also a prognostically important marker of structural heart disease. Importantly, imaging techniques that are used routinely for assessment of LVEF in cancer patients—echocardiography and MUGA scanning [72]—cannot assess cardiac fibrosis. CMR allows accurate detection and quantification of both reactive and replacement fibrosis [98, 127–129]—reactive fibrosis by T1 mapping and replacement fibrosis by delayed enhancement CMR (DE-CMR).
Two retrospective and one prospective studies from the group at the University of Manitoba described the presence of subepicardial delayed enhancement with a prevalence of 94–100 % in the context of cardiomyopathy in patients with breast cancer during [130, 131] and at the end of treatment [132] with anthracyclines and trastuzumab. However, subsequent studies from other groups [105, 133] have demonstrated that chemotherapy -related cardiotoxicity is not typically associated with any delayed enhancement during or early after treatment. In our collective experience, we have also observed no delayed enhancement in patients with cardiotoxicity during or soon after chemotherapy with either anthracyclines or trastuzumab.
Some patients with chronic cardiomyopathy from cardiotoxicity may develop delayed enhancement that is basal mid-myocardial or at the right ventricular insertion points [133]. The prevalence of these patterns is low [133–135]. These patterns are nonspecific and shared by chronic cardiomyopathies of any etiology. A study of 62 childhood cancer survivors treated with anthracyclines and imaged 7.8 years later described no delayed enhancement [136]. Thus, there is no pattern of delayed enhancement or replacement fibrosis that is unique to chemotherapy -related cardiotoxicity.
Since chemotherapy -related cardiotoxicity is not typically associated with replacement fibrosis, fibrosis seen on CMR with delayed enhancement imaging in the setting of a low LVEF could point to an alternative explanation for the cardiomyopathy, such as an ischemic cardiomyopathy or myocarditis [99]. Thus, delayed enhancement imaging is nevertheless useful in the evaluation of presumed chemotherapy -related cardiotoxicity to confirm the etiology of the cardiomyopathy and to rule out other diagnoses with important implications, such as ischemic heart disease .
CMR for Assessment of Concomitant Cardiac Disease in Cancer Patients
With the increasing number of older patients newly diagnosed with breast and other cancers [137], and as a result thereof the increasing prevalence of co-existing cardiovascular disease, timely diagnosis and initiation of treatment of these is essential prior to exposure to potentially cardiotoxic drug regimens. This is particularly important since preexisting cardiac conditions such as coronary artery disease or cardiomyopathy are known risk factors for both anthracycline- [55, 138] and trastuzumab-related [139] cardiac complications.
A CMR study is modular allowing the expansion of the basic CMR test protocol for LVEF assessment to include evaluation for ischemic heart disease and/or cardiomyopathy as indicated based on patients’ history of risk factors and symptoms. For the assessment of myocardial ischemia, there are few additional steps required, including intravenous access and contrast administration. Most elderly and cancer patients have limited exercise tolerance; therefore, pharmacological stress with vasodilators such as adenosine or regadenoson will be more practical in most cases. This “ischemia” protocol, which is typically combined with assessment of myocardial viability/scar with the delayed enhancement technique discussed above, adds approximately 20 min to the baseline exam without contrast. The diagnostic performance of stress CMR for detection of CAD in a recent meta-analysis was reported having a sensitivity of 91 % and specificity of 81 % [140]. Moreover, CMR was shown to be useful in women, who pose in general challenges for noninvasive CAD diagnosis due to smaller heart size, more frequent intermediate severity, and limited extent of CAD, with a sensitivity and specificity of 84 and 88 %, respectively [141].
The pericardium may be affected in cancer patients as the primary affected organ such as in pericardial mesothelioma, but this is rarely diagnosed clinically [142]. However, autopsy studies show that 19–40 % of patients dying of lung cancer, and 10–28 % dying of breast cancer have pericardial involvement, which is typically from direct spread of the primary chest tumor [143]. A sequela of pericardial tumor involvement is pericardial effusion, which can result in tamponade. Pericardial effusion can also be the cardiotoxic sequela of various anticancer agents [12]. Pericardial morphology and the presence of an effusion can be assessed by the same image data set obtained for LVEF assessment. These dynamic images also provide information on the hemodynamic relevance, if collapse of the right atrium and ventricle is present and if the inferior vena cava is dilated [144]. On delayed enhancement images obtained for myocardial tissue characterization, pericardial enhancement indicates the presence of inflammation. Pericardial thickening and typical findings of increased ventricular interdependence on dynamic cine imaging during respiration are hallmarks of constrictive pericarditis [145].
Strain Imaging by CMR
To better characterize the complex deformation and shortening of the helical myocardial layers during contraction, and to differentiate passive tethering of dysfunctional myocardial regions from active contraction, strain imaging was introduced [146, 147]. Strain is a measurement of the percent change in the fiber length and can be obtained in radial, circumferential, and longitudinal direction. A widely validated and reproducible tool for this purpose is tagged CMR, whereby noninvasive markers (e.g., tags) are placed in a grid-like format by locally induced perturbations of magnetization with selective radiofrequency saturation. Several motion quantification techniques are available; the most commonly used is harmonic phase (HARP) analysis [148]. Normal values for maximal longitudinal strain (%; mean ± SD) depend on age and gender and are highest at the apex and smallest at the base, ranging from −0.13 ± 0.04 to −0.15 ± 0.03 at the base to −0.18 ± 0.05 to −0.19 ± 0.04 at the apex [149]. One study assessed myocardial strain by speckle tracking echocardiography and CMR in childhood cancer survivors exposed to anthracycline therapy with normal systolic function. The authors found that both circumferential and longitudinal myocardial strains were reduced in these individuals compared to normal controls [average mid-peak circumferential strain magnitude −14.9 ± 1.4 versus −19.5 ± 2.1 (P < 0.001) and peak longitudinal strain magnitude −13.5 ± 1.9 versus −17.3 ± 1.4 (P < 0.001) by CMR] [150]. The added benefit of strain imaging is expected to be the detection of subclinical cardiotoxicity in patients receiving potentially cardiotoxic chemotherapies. To investigate changes in circumferential strain over the course of anthracycline therapy, Drafts et al. performed serial CMR imaging before and 1, 3, and 6 months after therapy in 53 patients. The authors found deterioration in circumferential strain (−17.7 ± 0.4 to −15.1 ± 0.4; p = 0.0003); interestingly this was accompanied by a likewise small but significant change in LVEF (58 ± 1 % to 53 ± 1 %; p = 0.0002) [105]. This small study raises a question needing further investigation about how much additional diagnostic information is gained by both imaging (strain, etc.) and non-imaging (troponin, etc.) tests over small changes in LVEF that can be detected by CMR as an indication for cardiotoxicity.
T1 and T2 Mapping by CMR to Characterize the Myocardium
T1 and T2 myocardial mapping are promising newer CMR techniques that offer a quantitative assessment of the myocardium (by using T1 and T2 relaxation times) in the evaluation of diffuse myocardial disease (fibrosis and edema).
Reactive fibrosis is most often assessed by CMR using the T1 mapping technique and is expressed using “native” T1 values when performed without contrast and as extracellular volume fraction (ECV) when performed with contrast. The longitudinal relaxation time T1 of a tissue indicates how rapidly protons recover after a radiofrequency pulse. Pre-contrast (or “native”) T1 varies with water content and may increase due to diffuse myocardial fibrosis, but it reflects a composite signal from both myocardial cells and the interstitium, and it varies with the measurement technique and CMR field strength. After gadolinium-based contrast administration, T1 times are shortened. The T1 times are a primary reflection of and are inversely proportional to the concentration of gadolinium in the interstitium. Thus, measuring T1 after contrast administration gives a better measure of the interstitial space. However, post-contrast T1 also varies with gadolinium dose, clearance rate, time after contrast administration, body composition, hematocrit, and the heart rate. If the change in T1 after contrast administration is measured in both the myocardium and blood after equilibration of the contrast distribution, the partition coefficient can be calculated. By correcting for the hematocrit level, the myocardial ECV is derived. The ECV is largely independent of the measurement technique and CMR field strength and is an inherent measure of the individual’s myocardial interstitial space.
T1 mapping has great potential for the evaluation of chemotherapy -related cardiotoxicity because the predominant type of fibrosis seen in cardiotoxicity is reactive fibrosis and not replacement fibrosis. Diffuse reactive fibrosis is, in fact, a hallmark of the condition. Animal studies of chronic anthracycline cardiotoxicity have shown higher native T1 values compared to controls [151, 152]. ECV has been shown to correlate with anthracycline dose, functional capacity, LV dysfunction, and markers of adverse LV remodeling in pediatric [153] and adult [154] patients after completion of anthracycline-based chemotherapy . Similarly, CMR measures of contrast-enhanced T1-weighted signal intensity were higher 3 months after initiating potentially cardiotoxic chemotherapy in a study of 65 cancer patients [155]. Thus, diffuse reactive fibrosis assessed by CMR has an important role in the pathophysiology and likely the prognosis of chemotherapy-related cardiotoxicity.
T2-weighted CMR is useful in distinguishing acute from chronic myocardial infarction according to the presence or absence of edema. However, T2-weighted sequences have a number of disadvantages including their susceptibility to artifacts and relatively lower differences in signal intensity between edematous and normal myocardium, making the image interpretation difficult. Also, similar to DE-CMR, T2-weighted imaging is based on identification of focally increased signal intensity compared to “normal remote” myocardium. Such a technique would not be useful to identify the presence of diffuse edema in the entire myocardium, as would be expected with chemotherapy -related cardiotoxicity. It is in this regard that T2 mapping would provide an objective and quantitative measure of diffuse myocardial edema.
In an animal study of early anthracycline cardiotoxicity, T2 values were increased in explanted hearts of anthracycline-treated rats in comparison to controls, even in the absence of LV dysfunction or histopathologic evidence of cardiac fibrosis or necrosis [156]. However, another rat study of anthracycline cardiotoxicity did not show any significant changes in T2 values in explanted hearts of anthracycline-treated rats [151]. In a human study of 65 cancer patients treated with anthracyclines who had CMRs before and 3 months after initiation of chemotherapy and had small but significant LVEF declines, there was no significant increase in myocardial relative enhancement (quantified as the ratio of myocardial to skeletal muscle signal intensity) on T2-weighted images [155]. There are three main explanations for the negative finding: one, there may be absence of significant edema with cardiotoxicity; two, this study only noted small declines in LVEF and edema may be seen in those with larger declines in LVEF; or three, imaging at 3 months may not be the right timing to detect edema from cardiotoxicity. Therefore, further research is warranted to investigate whether T2 mapping could be used for the early detection of cardiotoxicity.
Targeted Nuclear Imaging by CMR
Cell death by apoptosis is believed to be an important mechanism in ischemic and nonischemic cardiomyopathies [157]. An early event in apoptosis is translocation of phosphatidylserine on the extracellular surface of the cell membrane, where annexin V, a commercially available soluble protein, can bind. This protein has been recently conjugated to superparamagnetic iron oxide (SPIO) , which is a negative MRI contrast agent that can be visualized using a T2*-weighted MRI pulse sequence. Dash et al. have tested this technique to detect apoptosis in a mouse model of doxorubicin cardiotoxicity. They found a good correlation between CMR T2* signal loss and number of apoptotic cells in tissue samples. In vivo, they were able to detect apoptotic activity up to 10 days after doxorubicin exposure and moreover demonstrated that apoptosis is a reversible and treatable process with alpha-1-adrenergic receptor agonists [158].
Detection of Vascular Injury by CMR
In addition to HF from myocardial toxicity, damage to the endothelium and increased risk for vascular disease have been demonstrated after anthracyclines [59], hormone therapy [159], and therapies targeting the vascular endothelial growth factor (VGEF) pathway with bevacizumab [160] and sorafenib [161]. Using the phase-contrast velocity flow mapping technique, which allows the measurement of blood flow and the generation of flow velocity time curves , Chaosuwannakit et al. were able to demonstrate an increase in aortic stiffness after anthracycline therapy. They studied aortic blood flow in 40 patients before and after anthracycline treatment and demonstrated an increase in pulse wave velocities and decrease in aortic distensibility in treated patients but not in controls [59]. Endothelial function can be assessed noninvasively by flow-mediated arterial dilatation (FMAD) [162] and could be used in studies investigating vascular effects of chemotherapies.
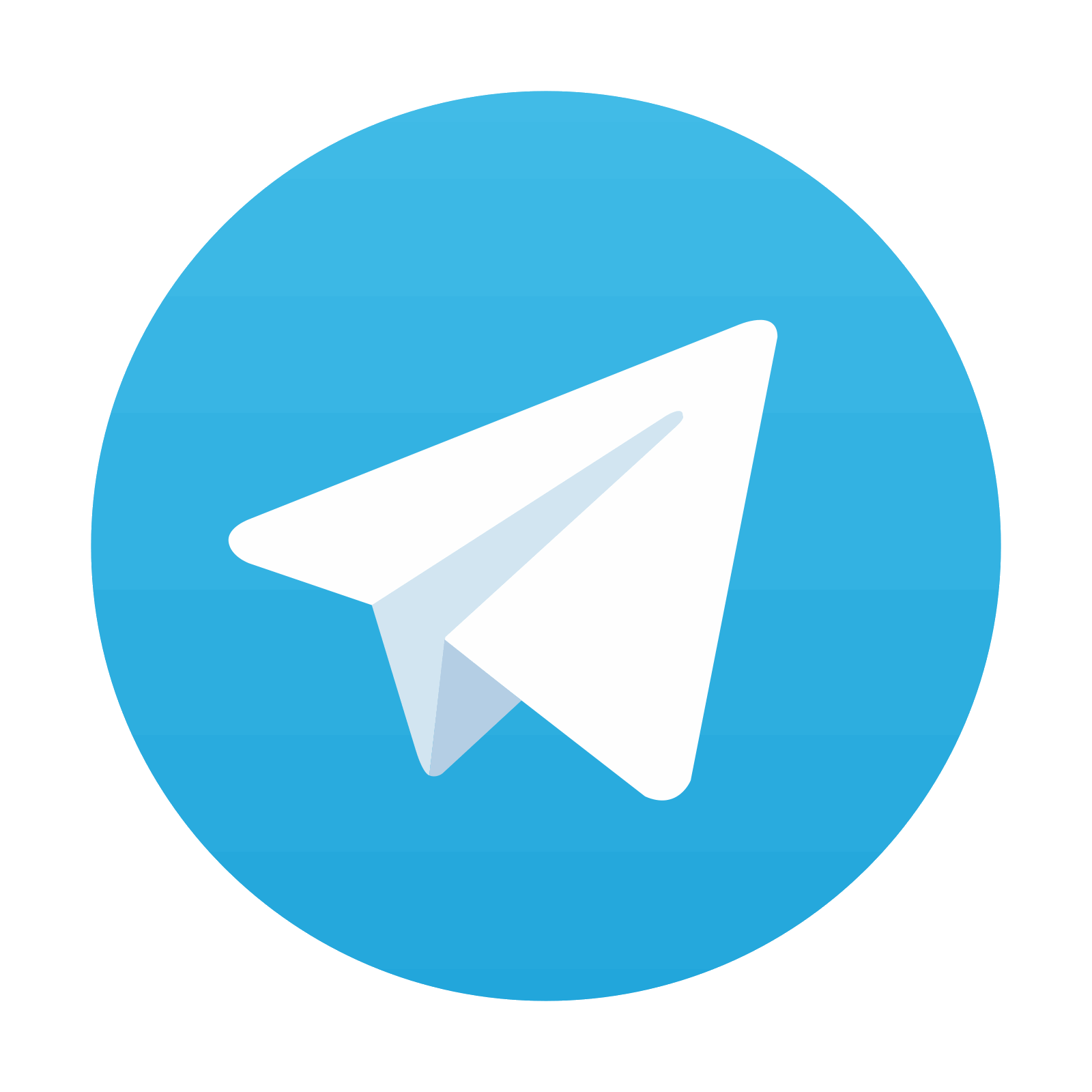
Stay updated, free articles. Join our Telegram channel
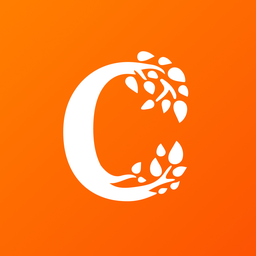
Full access? Get Clinical Tree
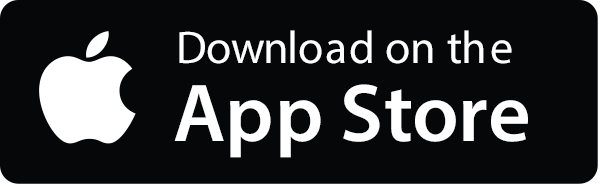
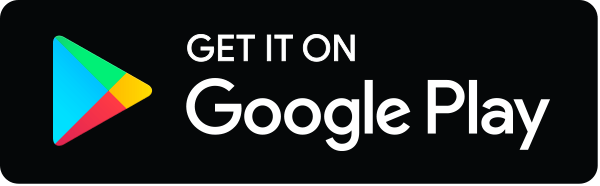
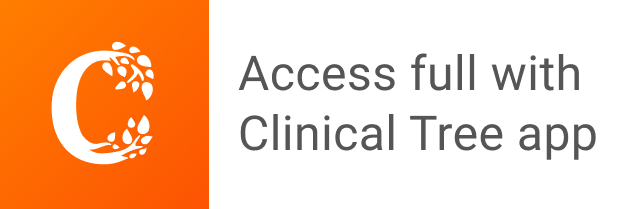