Fig. 8.1
Schematic structure of a sarcomere. Illustrated proteins are described throughout this chapter. Gray: Z-disc-associated proteins; lilac: proteins involved in mechanotransduction; brown: proteins involved in protein turnover and degradation; green: proteins associated with additional signaling pathways
Sarcomeres are aligned in series to allow force transmission from one to the next and finally to the sarcolemma. Z-discs of adjoining sarcomeres are connected via desmin, an intermediate filament (Frank and Frey 2011). At the lateral boundaries of the cell, Z-disc-associated proteins interact with a sarcolemmal protein complex termed costamere to ensure lateral anchorage and force transmission as well as signal transduction (Ervasti 2003).
Muscular contraction is generated by a sliding filament mechanism within the sarcomeres. Actin and myosin filaments slide along each other in an energy-consuming process, translating chemical energy into mechanic force. The combined action of all serially aligned sarcomeres leads to contraction of the muscle cell. The head part of myosin directly interacts with an actin filament. The neck part of myosin has the ability to perform a conformational change, which is the basis for a step-by-step movement of myosin along the actin filament. Each step requires the hydrolysis of ATP, is therefore energy consuming, and involves a detachment and reattachment of the myosin head to actin (Schmidt et al. 2004).
Tropomyosin and troponin are important regulatory proteins of the sliding filament mechanism (Kobayashi et al. 2008). Tropomyosin is a filamentary protein that is wound around actin filaments and inhibits the interaction between myosin and actin. In the event of a muscular contraction, a local increase of the calcium concentration leads to a conformational change of troponin. Since troponin is connected to tropomyosin, this leads to a displacement of tropomyosin and facilitates the interaction between myosin and actin.
The structural and force generating components of the sarcomere are closely connected to diverse intracellular signaling pathways. These interactions regulate the process of contraction, facilitate cellular adaption to different physiological and pathological stimuli, and link sarcomeric components to sarcolemmal structures as well as to the gene expression machinery within the nucleus. Of note, mutations in a multitude of sarcomeric proteins are known to cause familial cardiomyopathies (Frank et al. 2007; Kimura 2010; van Eldik and Passier 2013). The most important forms are hypertrophic cardiomyopathy (HCM) and dilated cardiomyopathy (DCM). Especially hypertrophic cardiomyopathy is in most cases based on a mutation in a sarcomeric protein. Most frequently, mutations are found in the genes coding for cardiac β myosin heavy chain, cardiac myosin-binding protein C, and cardiac muscle troponin T, but other sarcomeric proteins, including Z-disc proteins, may be involved as well (Frey et al. 2012).
In this chapter we will focus on the emerging role of the sarcomere as a hub for signal transduction in cardiomyocytes. First, we will describe how mechanotransduction, a process involving cellular sensing of mechanical stress, takes place at the level of the sarcomere. Next, we provide an outline on the regulation of degradation and the turnover of sarcomeric components. We will then discuss the modulation of sarcomeric proteins and their functional properties under conditions of oxidative stress. Finally, we will describe how eminent signaling pathways involving phosphatases and kinases are linked to the sarcomere.
8.2 Mechanotransduction
Mechanotransduction is the sensing of a mechanical stimulus and its conversion into a cellular response. A multitude of proteins have been shown to be involved in this process in cardiomyocytes. Sensing of mechanical stress is essential for cardiac adaption to hemodynamic load as well as for the induction of hypertrophic response in response to increased load due to different pathological and physiological conditions. The sarcomere at the interface between the contractile apparatus and the sarcolemma/costamere is ideally situated as a “hot spot” for proteins with a regulative function in mechanotransduction (Knoll et al. 2003; Frank et al. 2006, 2007; Buyandelger et al. 2014). Other mechanosensitive proteins are localized at the sarcolemma or in the cytosol (Sadoshima and Izumo 1997). Sarcomeric proteins are closely related to the dynamic structures of the contractile apparatus. They may sense mechanical stress and activate intracellular signaling pathways, which coordinate a cellular response. The highly structured protein complexes of the Z-disc and the M-band are centrally involved in the process of mechanotransduction (Gautel 2011). Mechanical stress induces profound alterations in gene cardiomyocyte expression (Frank et al. 2008). Some well-characterized proteins with mechanosensitive functions are discussed below.
8.2.1 Titin and Associated Signaling Pathways
The giant protein titin is one of the main structural components of the sarcomere. Titin acts as a scaffolding protein for myofibrillar assembly, serves as molecular ruler for the tick filaments, fixes the position of the myosin filaments to the middle of the sarcomere, and is a molecular spring, which regulates sarcomeric elasticity. Different titin isoforms vary in their stiffness. The elastic elements of titin are located in the I-band region. The properties of the molecular spring can be fine-tuned by phosphorylation at different sites (Kruger and Linke 2011), and as recently demonstrated, also by S-glutathionylation of cryptic cysteine residues (Alegre-Cebollada et al. 2014). Of note, the latter mechanism appears to be a general mechanism to regulate tissue elasticity.
Proteins from the four-and-a-half LIM domain family are highly expressed in cardiac and skeletal muscle. Four-and-a-half LIM protein 1 (FHL1) is upregulated in the context of pathological cardiac remodeling (Chu et al. 2000). FHL1 binds to titin at the N2B domain in the I-band region. FHL-1 knockout mice exhibit a reduced hypertrophic remodeling after transverse aortic constriction, which is a strong mechanical stress stimulus. The connection between FHL1 and an elastic part of titin indicates a distinct function in mechanotransduction. In addition, FHL1 acts as a scaffold protein for components of the MAP-kinase signaling pathway. Thus, FHL1 provides a link between this important pathway involved in cardiac hypertrophic remodeling and the sarcomeric protein titin (Sheikh et al. 2008). Finally, FHL1 mutations have been shown to cause hypertrophic cardiomyopathy in humans (Friedrich et al. 2012). Interestingly, this also involves impaired proteasome function, implying a pathophysiological link between altered mechanotransduction and protein degradation. Moreover, several members of the muscle ankyrin repeat protein (MARP) family interact with titin at its elastic regions. Upon mechanical stretch, MARPs show an intracellular redistribution. Thus, MARPs are part of a mechanosensitive element (Miller et al. 2003). Proteins of the MARP family are thought to be another key part of the stress response system in muscles, since they are induced under different pathological conditions like starvation, stretch, injury, or denervation.
Finally, titin also contains a kinase domain, which is located near the M-line. Titin kinase is activated by mechanical stretch, also linking this enzymatic domain to mechanotransduction (Puchner et al. 2008). Titin kinase interacts with a protein complex containing nbr1, p62, and MURF2. Upon mechanical inactivity, MURF2 translocates to the nucleus, where it serves as an interaction partner of serum response factor (SRF). This pathway provides a direct link between mechanosensing and altered gene expression and thereby contributes to the development of atrophy (Lange et al. 2005).
8.2.2 Telethonin/T-Cap
Telethonin, also called T-cap, is a sarcomeric Z-disc protein that binds to the N-terminal part of titin (Zou et al. 2006). In addition to that, telethonin has several other binding partners like the calsarcins, myostatin, or ion channels (Frank et al. 2006). Telethonin provides a link between mechanical stress sensing and apoptosis. Telethonin influences the function of p53, a key regulator of the cell cycle and tumor suppressor gene, and thus connects the sarcomeric Z-disc to the regulation of apoptosis. Telethonin knockout mice do not exhibit a cardiac pathology spontaneously. However, under conditions of mechanical stress, caused by transverse aortic constriction, an increase in apoptosis is evident, which might contribute to the development of heart failure (Knoll et al. 2011).
8.2.3 Muscle Lim Protein
Muscle Lim protein (MLP) is highly expressed in striated muscle tissues. Besides other binding partners at different cellular structures, MLP interacts with sarcomeric proteins like α-actinin and telethonin/t-cap (Knoll et al. 2002; Heineke et al. 2005; Frank and Frey 2011). MLP-deficient mice develop a severe cardiomyopathic phenotype (Arber et al. 1997). MLP has been proposed to be a component of a mechanical stretch sensor. Mechanical stretch is accompanied by increased expression of BNP, a marker protein of hypertrophic remodeling. In cardiomyocytes from MLP knockout mice, the induction of BNP expression after mechanical stretch is markedly blunted while the response to pharmacological stimuli remains unaltered (Knoll et al. 2002). Mechanical strain and pharmacological stimulation of cardiomyocytes lead to nuclear translocation of MLP. This observation also applies to rat hearts that were subjected to aortic banding or myocardial infarction (Boateng et al. 2007, 2009). In addition to its function as a nucleoplasmatic shuttling protein in the context of hypertrophic remodeling, MLP is also associated with the calcineurin pathway (see below). However, the exact cardiac function of MLP is not completely understood and controversially discussed. As described above, compelling data support a role for MLP in the regulation of cardiac remodeling. Yet, MLP transgenic mice do not exhibit a pathological cardiac phenotype. In addition to that, the extent of cardiac remodeling as a reaction to different pathological stimuli like aortic constriction or infusion of angiotensin II is equal in MLP transgenic and wild-type mice (Kuhn et al. 2012).
8.2.4 LRRC39/Myomasp
LRRC39/Myomasp is a novel M-band protein with a potential function in mechanical stress sensing (Will et al. 2010). It exhibits a strong enrichment in cardiac and skeletal muscle. Myomasp localizes to the sarcomeric M-band in cardiomyocytes and directly binds to the thick filament protein myosin heavy chain. Mechanical stretch of cardiomyocytes leads to a reduced expression of myomasp, indicating a connection to mechanosensation. This is further supported by a marked cardiac downregulation of myomasp in mice that underwent transverse aortic constriction, which causes mechanical strain in vivo. Furthermore, a knockdown of myomasp is associated with reduced expression of other structurally important M-band-bound proteins like myosin heavy chain or myomesins 1 and 2, as well as an impaired contractile function. These alterations can partly be explained by a reduction of SRF-dependent gene expression. On the other hand, a knockdown of myomasp induces an enhanced expression of typical stretch responsive marker proteins like BNP and GDF15, which generally denotes an activation of stress-associated pathways. A knockdown of the myomasp orthologue in zebrafish leads to contractile dysfunction, underscoring the importance of an intact M-band structure for proper sarcomeric function.
8.3 Protein Degradation and Turnover
An exact equilibrium between protein synthesis and protein degradation is essential for the maintenance of cardiac function. This principle applies in particular to sarcomeric proteins, which have to be assembled in a precise stoichiometry to build the backbone of the cardiomyocyte’s force generation. The degradation of sarcomeric proteins is thus tightly regulated and specific mechanisms for the degradation of certain components have been elucidated (Lyon et al. 2013).
Protein degradation is mainly performed by the ubiquitin-proteasome system, by autophagy, and by the calpain system. These systems provide the basis to recycle or degrade misfolded, unused, or damaged proteins. They have complementary functions, as for example the autophagy system is able to handle larger components like protein aggregates and even entire organelles (Portbury et al. 2011).
The ubiquitin-proteasome system consists of three classes of enzymes, which are necessary to select a protein for degradation and mark it with ubiquitin. In the first step an ubiquitin monomer is activated by an E1 enzyme in an ATP-dependent process. The second step is the transfer of ubiquitin to an E2 enzyme. Finally, an E3 enzyme transfers the ubiquitin moiety from the E2 enzyme to a selected protein and hereby marks it for degradation. E3 enzymes, which are also called ubiquitin ligases, ensure substrate specificity of the ubiquitin-proteasome system (Pickart 2001). Additional ubiquitin monomers bind to the ubiquitinated protein and form an ubiquitin chain. After that, the ubiquitinated protein is disassembled by a proteasome unit. The amino acids set free in the degradation process are substrates for the synthesis of new proteins. Several ubiquitin ligases are expressed in cardiomyocytes and some are expressed in a heart- and/or skeletal-muscle-specific fashion, implying an important role of this machinery in contractile cells. Not surprisingly, different cardiac pathologies have been linked to an altered function of components of the ubiquitin-proteasome system (Willis et al. 2014). Impairment of the ubiquitin-proteasome system is involved in the pathogenesis of inherited cardiomyopathies like HCM and DCM (Schlossarek et al. 2014). A connection between ubiquitin-proteasome impairment and HCM is, for example, well characterized for mutations in the MYBPC3 gene, coding for myosin-binding protein C. Mutations in MYBPC3 frequently lead to the formation of a truncated protein (Schlossarek et al. 2011). These mutated proteins are predominantly degraded by the ubiquitin-proteasome system. The excessive utilization of this system may cause its inability to ensure an adequate protein turnover, which potentially contributes to the pathogenesis of HCM (Sarikas et al. 2005). Mice with a knock-in of a truncated version of MYBPC3 exhibit a phenotype of cardiac hypertrophy and show an impairment of the ubiquitin-proteasome system with increasing age (Schlossarek et al. 2012).
Autophagy is another mechanism for the degradation of different cellular components, including sarcomeric proteins. Selected substrates are coated by a membrane. These vesicles then fuse with a lysosome, which contains acid hydrolase enzymes (Portbury et al. 2011; Lyon et al. 2013). Autophagy is required for a regular cardiac structure and function. Knockout of Atg5, a central component of the autophagy system, in mice leads to cardiomyopathy. This phenotype is associated with disarrangements of sarcomeric structures and a morphological disorganization of mitochondria (Nakai et al. 2007). In humans, defective autophagy due to mutations in the autophagy regulator EPG5 has been shown to cause Vici syndrome, a multisystem disorder with severe cardiomyopathy (Cullup et al. 2013). Autophagy and the ubiquitin-proteasome system are connected, so that ubiquitinated proteins may also be degraded by transferring them to the lysosome (Kirkin et al. 2009).
In the following we will focus on cardiac-enriched proteins and their potential role in cardiomyocyte protein turnover as well as cardiac (patho)physiology.
8.3.1 Muscle-Specific RING Finger Proteins
Muscle-specific RING finger (MuRF) proteins are a group of muscle-specific ubiquitin ligases. Their function in protein degradation and turnover has been studied in cardiac and skeletal muscle. Besides other cellular compartments, MuRF proteins localize to the sarcomeres and bind to several sarcomeric proteins (McElhinny et al. 2004; Witt et al. 2005). MuRF1 binds to the sarcomeric protein titin in the M-band region (Centner et al. 2001). It regulates the degradation of the thin filament-associated protein troponin I in cardiomyocytes. Overexpression of MuRF1 in cardiomyocytes in vitro is associated with changes in the contractile properties of the cells (Kedar et al. 2004). MuRF3 regulates the turnover of the sarcomere-associated proteins γ-Filamin and of four-and-a-half LIM domain protein 2 (FHL2) (Fielitz et al. 2007). Several in vivo studies in mouse models point to a function of MuRF proteins in the regulation of cardiac and skeletal muscle mass. MuRF1 and MuRF2 seem to have some functional redundancy in this regard, since MuRF1 and MuRF2 knockout mice develop normally, while double knockout mice exhibit severe cardiac and skeletal muscle hypertrophy (Witt et al. 2008). Conversely, MuRF1 transgenic mice have thin left ventricular walls and a reduced contractile performance (Willis et al. 2009b). MuRF1-mediated protein degradation thus seems to be an important mechanism in the process of cardiac atrophy (Willis et al. 2009a). Certain mutations in the TRIM63 gene, coding for MuRF1, lead to impaired protein degradation and have been identified in patients with HCM. Underscoring the pathophysiological relevance, significant cardiac hypertrophy is observed in mice expressing a mutated variant of MuRF1 (Chen et al. 2012).
8.3.2 F-Box and Leucine-Rich Repeat Protein 22
F-Box and Leucine-Rich Repeat Protein 22 (Fbxl22) is a recently described ubiquitin ligase with important functions regarding the turnover of cardiac sarcomeric proteins (Spaich et al. 2012). Fbxl22 exhibits a highly cardiac-enriched expression pattern. On the subcellular level, Fbxl22 localizes to the sarcomeric Z-disc. Components of a known ubiquitin ligase complex such as cullin and SKP1 are direct binding partners of Fbxl22. Protein degradation of two major sarcomeric proteins, α-actinin and γ-filamin (Filamin C), by the proteasome is mediated via the specific ubiquitin ligase function of Fbxl22. Conditions of cardiac stress, which typically cause cardiac hypertrophy, lead to a reduced expression of Fbxl22. Targeted knockdown of Fbxl22 in zebrafish embryos induces a severe cardiomyopathic phenotype, again underscoring the fundamental importance of a tightly regulated turnover of sarcomeric proteins.
8.3.3 Atrogin-1
Atrogin-1 is another muscle-specific protein from the group of F-Box proteins. Together with the cullin-RING protein cullin 1 and other proteins, atrogin-1 forms an E3 ubiquitin ligase complex. Atrogin-1 has the function of a substrate-specific adaptor protein (Lyon et al. 2013). It localizes to the sarcomeric Z-disc and directly binds to α-actinin-2 (Li et al. 2004). Until now, there is no evidence for a role of atrogin-1 in the mediation of protein turnover of sarcomeric proteins under physiological conditions. However, atrogin-1 provides a link to the phosphatase calcineurin, a prominent part of a key pathway of cardiac hypertrophy, which is associated with the sarcomeric Z-disc. Atrogin-1 directly binds to calcineurin at the Z-disc and mediates its degradation via the ubiquitin-proteasome system. When overexpressed, atrogin-1 hereby represses the development of cardiac hypertrophy as a reaction to different pathological stimuli. In addition to that, atrogin-1 regulates the ubiquitination of a truncated mutant of the thick filament-associated protein myosin-binding protein C, which is known to cause familial hypertrophic cardiomyopathy (Mearini et al. 2010).
8.3.4 Calpains
The calpains form a group of Ca2+-dependent proteases. Their proteolytic functions are involved in the degradation of sarcomeric proteins in cardiac and skeletal muscle (Portbury et al. 2011). Doxorubicin induces a calpain-mediated degradation of the sarcomeric protein titin (Lim et al. 2004). Cardiac troponins are substrates for proteolysis by calpains as well (Ke et al. 2008). Calpains and the ubiquitin-proteasome system act in concert in the degradation of sarcomeric proteins. Overexpression of calpain-1 in cardiomyocytes leads to a degradation of specific proteins and is accompanied by an increase in protein ubiquitination. Calpain-1 transgenic mice exhibit an enhanced cardiac protein ubiquitination and proteasome activity. Depending on the level of overexpression in these mice, increased lethality is observed, which is associated with the occurrence of characteristics of heart failure (Galvez et al. 2007).
Taken together, the turnover of sarcomeric proteins is cooperatively coordinated by several protein degradation systems. Malfunctions in components of these systems result in disturbed protein homeostasis, which contributes to the pathogenesis of cardiomyopathies.
8.4 Oxidative Stress
Oxidative stress is characterized by an increased formation of reactive oxygen species (ROS) like hydrogen peroxide. It occurs in different cardiac pathologies. ROS interfere with normal cellular functions either by directly damaging proteins and other components or by activating ROS-sensitive enzymes. ROS modify sarcomeric proteins and hereby influence their proper function. In addition to that, several ROS-sensitive enzymes with sarcomeric targets are activated during oxidative stress (Sumandea and Steinberg 2011).
In the presence of NO during oxidative stress formation of peroxynitrite occurs. Peroxynitrite is a highly reactive compound that can induce nitration of tyrosine residues. ROS usually react with thiol moieties of cysteines. Especially cysteines flanked by aromatic or basic amino acids are susceptible to interactions with ROS. Modified residues within the amino acid sequence can lead to conformational changes of a protein or form bonds with other residues (Steinberg 2013). Overall, ROS modifications due to oxidative stress induce extensive changes in cellular functions. ROS usually modify diverse targets and sometimes at different sites. The type of oxidant species and the level of oxidative stress influence the cellular responses.
8.4.1 Direct Modification of Sarcomeric Proteins
ROS lead to changes in contractile function partially by direct modification of sarcomeric proteins. ROS modifications of myosin induce changes in protein structure and inhibit the activity of its ATPase in the head domain (Tiago et al. 2006; Passarelli et al. 2008). In context of ischemia/reperfusion injury, oxidative modifications of actin and tropomyosin occur (Canton et al. 2004). Glutathionylation of actin leads to a reduced myosin head ATPase activity and influences the dynamics of the actin–myosin binding (Pizarro and Ogut 2009). Disulfide cross-bridge formation in tropomyosin as a result of oxidative stress is associated with contractile dysfunction (Canton et al. 2006). ROS modifications also increase the stiffness of titin (Grutzner et al. 2009). Thus, passive elasticity of the sarcomere is reduced under conditions of oxidative stress. While most ROS modifications of sarcomeric proteins lead to a depressed contractile function, nitroxyl (HNO) has an opposite effect. HNO-induced modifications of myofilaments increase their Ca2+ responsiveness and enhance force generation (Gao et al. 2012).
8.4.2 Modification of Sarcomeric Proteins by ROS-Sensitive Enzymes
Several kinases involved in this process of posttranslational modifications are ROS-sensitive enzymes. Oxidative stress generally increases protein phosphorylation by activation of kinases and simultaneous inhibition of phosphatases. Particularly titin, certain subunits of troponin and the thick filament-associated protein (cMyBP-C) are phosphorylated by ROS-activated kinases (Sumandea and Steinberg 2011; Steinberg 2013).
Ca2+ sensitivity and functional properties of troponin I are modulated via phosphorylation by protein kinase A, CβII, Cδ, D, and p90 ribosomal S6 kinase (Haworth et al. 2004; Itoh et al. 2005; Brennan et al. 2006; Wang et al. 2006b; Sumandea et al. 2008). Protein kinase A (Type I) contains ROS-sensitive residues within its regulatory subunits. Modification of these sites by hydrogen peroxide induces formation of a disulfide bond and a translocation to myosin heavy chain, which functions as an A kinase anchoring protein (AKAP). This enhances phosphorylation of troponin I and cMyBP-C at the sarcomere by protein kinase A independently of the usual β-adrenoceptor-associated pathway. In consequence, oxidative stress enhances contractility (Brennan et al. 2006). Protein kinase Cδ can be activated in a hydrogen peroxide-induced mechanism that includes a certain phosphorylation by Src kinases. In this context, oxidative stress leads to a lipid-independent activation of protein kinase Cδ (Rybin et al. 2004). Protein kinase D is activated by hydrogen peroxide via a protein kinase C-dependent pathway (Waldron and Rozengurt 2000). However, activation of protein kinase D under conditions of oxidative stress is associated with a translocation of this enzyme to the nucleus, while an enhanced phosphorylation of sarcomeric proteins is unclear (Waldron et al. 2004).
Troponin T is phosphorylated by Raf-1, protein kinase Cα, or apoptosis signal-regulating kinase-1 (ASK-1), which is a ROS-sensitive enzyme involved in the regulation of apoptosis (He et al. 2003; Sumandea et al. 2003; Yamaguchi et al. 2003; Pfleiderer et al. 2009). Phosphorylation of tropronin T by protein kinase Cα is associated with a decreased Ca2+ sensitivity of the myofilaments, a diminished myosin ATPase activity, and a reduced contractility (Sumandea et al. 2003). ROS-induced activation of ASK-1 leads to contractile dysfunction. However, besides increased phosphorylation of the sarcomeric protein troponin T, changes in calcium handling may be a different explanation for this effect (He et al. 2003). In summary, phosphorylation of sarcomeric proteins by ROS-induced kinases has a significant effect on cardiomyocyte contractility.
8.4.3 ROS-Induced Cleavage of Sarcomeric Proteins
Oxidative stress leads to an increased cleavage of sarcomeric proteins, which may contribute to contractile dysfunction. Ischemic injury induces a degradation of sarcomeric proteins, including troponin I, troponin T, and myosin light chain 1 (MLC-1) (Van Eyk et al. 1998; Zhang et al. 2006). These protein cleavages are linked to an activation of the calpain system (Steinberg 2013). Matrix metalloproteinase 2 (MMP-2) is usually excreted to the extracellular matrix and cleavage of MMP-2 by other MMPs leads to its activation. MMP-2 has important functions in extracellular tissue remodeling. However, MMP-2 is not exclusively secreted from the cardiomyocytes but to some extent remains intracellular (Ali et al. 2012). MMP-2 serves as a central mediator of ROS-induced sarcomeric protein degradation, which contributes to impaired contractility (Ali et al. 2011). Overexpression of MMP-2 leads to reduced contractility and decreased responsiveness to inotropic stimuli (Wang et al. 2006a). The proteolytic activity of MMP-2 is initially inhibited by an interaction between a cysteine residue in the propeptide domain and the catalytic domain (Steinberg 2013). MMP-2 can be activated by ROS-induced modifications at this cysteine residue (Viappiani et al. 2009). Ischemia/reperfusion injury leads to a degradation of MLC-1, MLC-2, troponin I, and titin by MMP-2 (Wang et al. 2002; Sawicki et al. 2005; Ali et al. 2010; Doroszko et al. 2010). In the context of oxidative stress, MMP-2 also cleaves the sarcomeric Z-disc protein α-actinin (Sung et al. 2007).
8.5 Phosphatases and Kinases
Phosphatases and kinases are key enzymes that regulate cellular functions via phosphorylation or dephosphorylation of target proteins. A plethora of intracellular signaling pathways are controlled by phosphatases and kinases. Of note, several phosphorylation cascades are also integrated in signaling pathways that connect sarcomeric structures to other compartments of the cardiomyocyte, in particular the nucleus. Specifically, the function of many sarcomeric proteins, including titin, myosin-binding protein C, myosin regulatory light chain, and troponin subunits, is modulated by phosphorylation (James and Robbins 2011; Kamm and Stull 2011; Solaro and Kobayashi 2011; Hidalgo and Granzier 2013).
< div class='tao-gold-member'>
Only gold members can continue reading. Log In or Register a > to continue
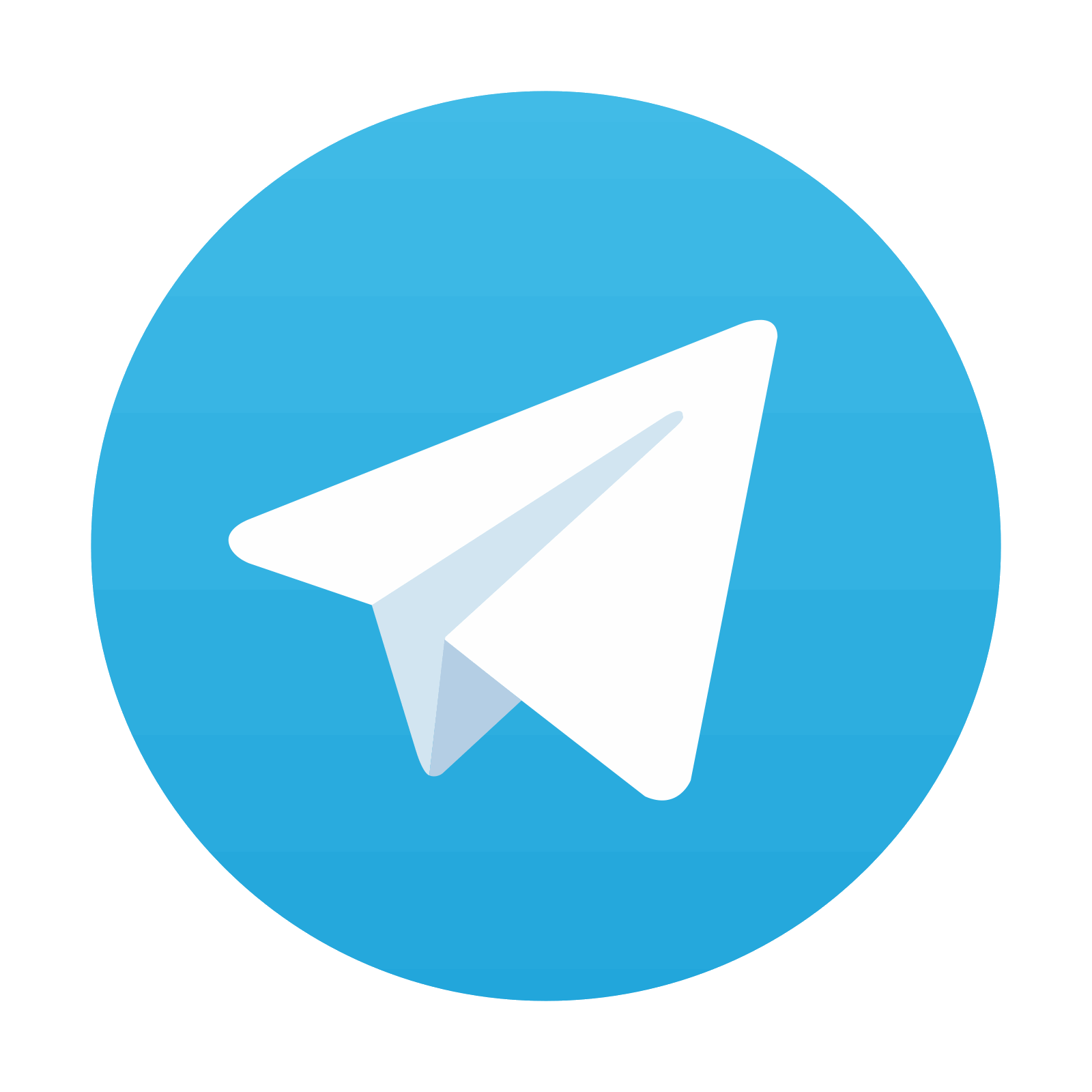
Stay updated, free articles. Join our Telegram channel
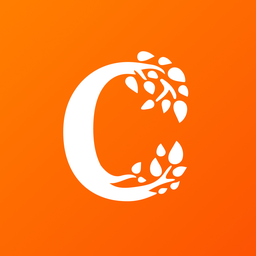
Full access? Get Clinical Tree
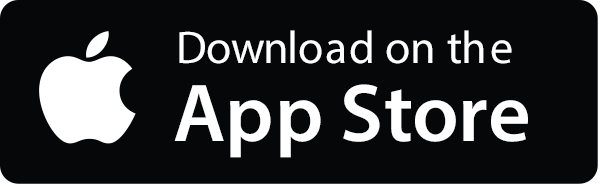
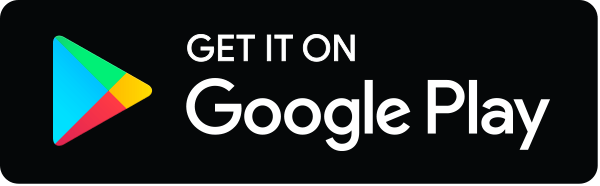