Fig. 1.1
Concept of automated soft tissue dissection. A standard industrial robot is used to hold a high frequency ultrasound probe. The probe detects the flow-signal of the vessel (i.e. internal thoracic artery) and automatically follows the signal along the path of the vessel. This information can be used to guide a second robot that guides a dissecting tool and dissects the soft tissue within a predefined safety margin along the path of the vessel. Joint Project of the German Aerospace Center (DLR) and the Universities of Leipzig and Zurich funded by the Deutsche Forschungsgemeinschaft (DFG)
Lokomats
The Lokomat is a bilateral robotic orthotic that is used in conjunction with a body-weight support system to control patient leg movements in the sagittal plane [10]. The Lokomat’s hip and knee joints are actuated by linear drives, which are integrated in an exoskeleton structure. A passive foot lifter induces an ankle dorsiflexion during the swing phase. The legs of the patient are moved with highly repeatable predefined hip and knee joint trajectories on the basis of a position control strategy. Knee and hip joint torques can be determined from force sensors integrated inside the Lokomat (Fig. 1.2). Novel control strategies enable the use of the device in an “assist as needed” manner in order to maximize patient participation [11].
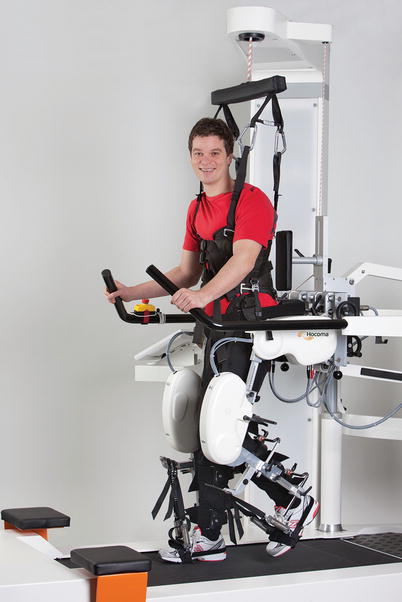
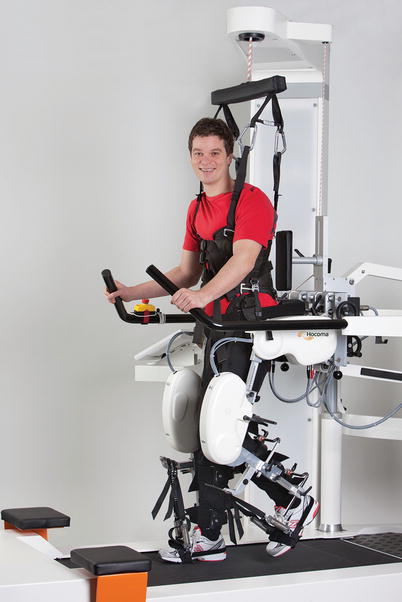
Fig. 1.2
The rehabilitation robot Lokomat is a bilateral robotic orthosis that is used in conjunction with a body-weight support system to control patient leg movements (Copyright Hocoma; used with permission)
ARMin is another example of an exoskeleton structure. The latest prototype (ARMin III) has seven independent degrees of freedom (DOF) allowing 3D shoulder rotation, elbow flexion/extension, pro/supination of the lower arm, wrist flexion/extension, and hand opening/closing. A special shoulder module was developed that takes into account the fact that the center of rotation of the shoulder moves in vertical direction when the arm is lifted. This function is required to provide an anatomically correct shoulder movement and to avoid shoulder stress when lifting the upper arm above face level from misalignment of the robot and anatomical joint axes. The device allows passive mobilization, active game-supported arm therapy, and training of activities of daily living. For passive mobilization, a teach-and-repeat procedure has been implemented, where the therapist can move the patient’s arm on an arbitrary but patient-specific trajectory, while the robot is friction and gravity-compensated. The recorded movement can be repeated by the robot, while the patient is instructed to behave passively (relaxed, limp). In game mode, ARMin serves as an input device driving a graphical object (e.g., ball, token, cursor) in a computer game (e.g., ping-pong). In the ADL training mode the patient has to solve an ADL task presented by the audiovisual display (e.g., filling a glass and drinking). Key features in the game and ADL modes are different patient-responsive strategies that support the patient only when necessary.
Currently, Lokomats are used exclusively for orthopaedic and neuro-rehabilitation. A first pilot project has started at the University of Zürich to explore the usefulness of Lokomats to support cardiac rehabilitation in end-stage heart failure patients. Potential applications could also include rehabilitation of patients with general weakness and severe muscle loss after long-term intensive care treatment.
Robot-Assisted Radiosurgery
The CyberKnife Robotic Radiosurgery System uses image-guidance and computer controlled robotics to treat tumors by delivering multiple beams of high-energy radiation. Superior targeting accuracy is achieved by continuous image guidance and automatic movement corrections. The linear accelerator is mounted to a robotic arm that moves around the patient following a 4-D treatment plan. Radiation doses are limited to intended target while maximizing healthy tissue preservation. Motion compensation is provided using fiducial-free soft tissue tracking. For radiation of lung tumors a frameless respiratory tracking system accounts for tumor and surrounding structure motion as well as deformation throughout the entire breathing cycle. As a result contoured beams can be delivered precisely compensating for breathing induced tumor motion (Fig. 1.3). More recently, the system has been applied in animal arrhythmia experiments for non-invasive stereotactic robotic radiosurgery for remote ablation of the cavo-tricuspid isthmus, AV node; the pulmonary vein- left atrial junction, or the left atrial appendage. In this experiment it was feasible to produce bidirectional cavo-tricuspid isthmus block and AV nodal conduction block. The pulmonary vein-left atrial junction and left atrial appendage showed marked reduction in voltage. No evidence of radiation damage outside the target was found, demonstrating the feasibility of non-invasive treatment to create cardiac lesions [12].
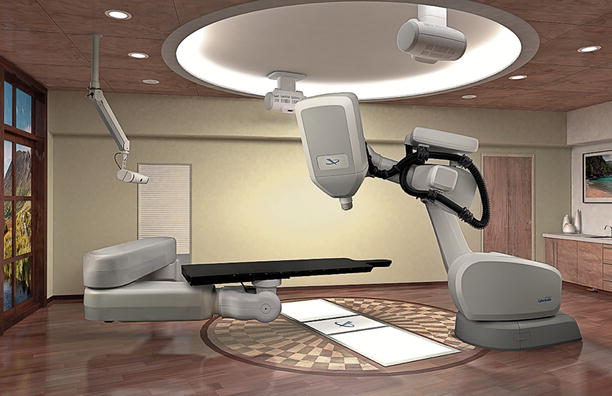
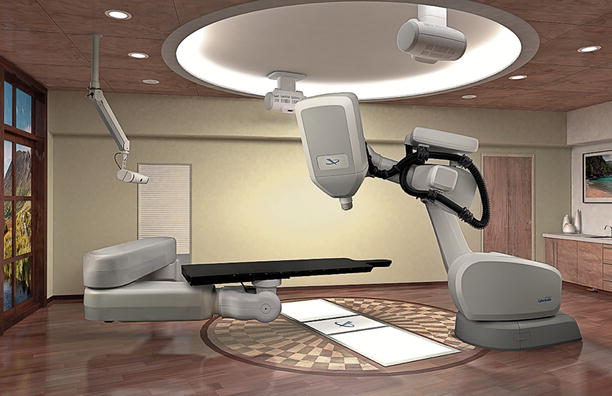
Fig. 1.3
Robot mounted linear accelerator (Cyberknife) for radiation treatment (Copyright Accuray, Inc., used with permission)
Robot Driven Fluoroscopy
With the advent of trans-catheter valve implantation, hybrid operating rooms have become popular in cardiac surgery. While standard fluoroscopy units use traditional C-arm technology, more recently robotic arms are used to position and orient the fluoroscope (Artis Zeego, Siemens Erlangen Germany). This system maintains projection during gantry rotation and maintains the iso center. Moreover, the system tracks the table and facilitates rotational angiography.
Surgical Tele-manipulators
Limitations of Manual Endoscopy
With the introduction of endoscopic techniques in the early 1980s, minimally invasive surgery became established broadly in numerous fields. It was not until the mid-1990s that the cardiac surgical community accepted minimally invasive techniques. Working in a confined space, such as the closed chest, presents the human operator with the challenge of a distant work environment that requires microsurgical precision. Technical and anatomical limitations as well as human factors complicate this complex interaction. Working through ports decreases surgical trauma significantly but limits the available access to perform complex motions and substantially impairs operator dexterity (see Appendix). Most endoscopic instruments provide only 4° of motion freedom at the tip of the instrument. This severely impairs the surgeon’s ability to impart dexterous tip motion. As a consequence, standard endoscopic instruments often do not allow free orientation of the instrument tip relative to the tissue (Fig. 1.4). The fulcrum effect adds the problem of reverse motion when using conventional endoscopic tools. Since standard endoscopic instruments pivot around a fixed entry point in the body, scale is largely dependent on the ratio of internal and external shaft length. Due to the resistance of the body wall, shear stress is generated at the pivot point, leading to a non-linear force relationship between the handle and the tip of the instrument. Not only does this increase operator fatigue but also leads to a lack of force feedback. Another key limitation of endoscopic surgery is the loss of hand-eye coordination. Normally, the endoscope enters a port in the body that is not collinear with the surgeon’s eyesight, thus forcing the surgeon to learn how to operate the tools from a perspective different than seen with his or her eyes. Using a single camera scope prevents stereovision and impairs depth perception severely, which in turn impacts upon the execution of targeted motions. In addition, active assistance, an indispensable component in open surgery, is almost impossible in thoracoscopic procedures. The transition from limited access cardiac or thoracic surgery to endoscopic surgery therefore aggravates procedure efficiency substantially. As a result previous attempts to perform endoscopic cardiac surgery using conventional endoscopic instruments have failed most surgeons. Surgical tele-manipulators were thought to overcome some of these limitations [13, 14].
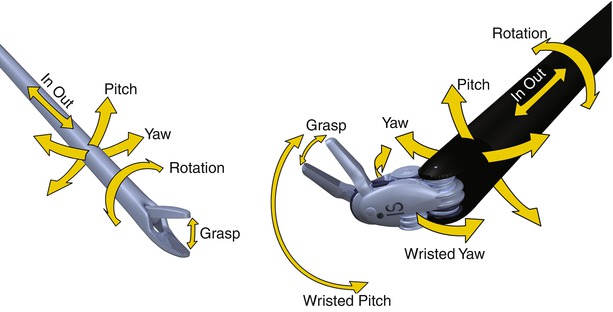
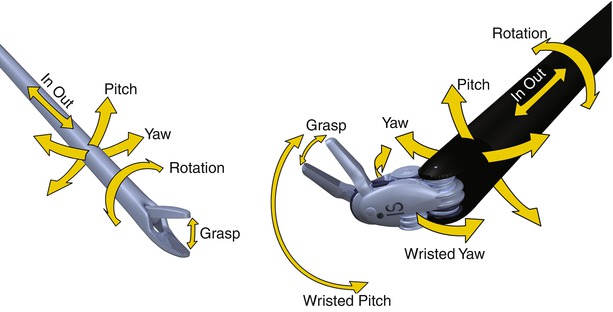
Fig. 1.4
Right: Principle of wrist motion of standard laparoscopic instrumentation (4° of freedom). Left: Principle of wrist motion of the da Vinci system. Six degrees of freedom are provided internally and allow free orientation and positioning in 3D-space (Copyright Intuitive Surgical, Inc., used with permission)
Principles of Telemanipulation Technology
The development of surgical tele-manipulators was initially not driven by the shortcomings of endoscopic instruments but stimulated by the idea of remote care of trauma patients. An intuitive tele-manipulator system that would allow distant surgeons to treat injured patients remotely was thought to possibly improve outcomes from severe injuries. As a result, a prototype tele-presence surgery system [SRI International, Menlo Park, CA] with bimanual force-reflective manipulators with 4° of freedom [DOF, see Appendix], interchangeable surgical instruments, and stereoscopic video input was developed and tested to treat remotely typical war injuries such as vessel tears and lacerations of internal organs [15, 16]. In parallel, the German Research Institute in Karlsruhe developed the first six DOF tele-manipulator (Advanced Robot and Telemanipulator System for Minimally Invasive Surgery, ARTEMIS). This platform was the first to be designed to improve dexterity during laparoscopic procedures and was used successfully to perform remote tele-operated cholcystectomies in pigs [17].
There are three key components that comprise tele-manipulator systems such as the Intuitive Surgical Da Vinci™ System (Fig. 1.5). The first two components, a master and remote manipulator pair, provide kinesthetic coupling between the human operator and the remote environment. This coupling implies that force commands from the human are replicated at the environment site and forces generated by the interaction at the surgical site are reflected back to the operator. This force coupling is essential to enable the operator to perform physical work in the distant environment in a manner that is intuitive and productive. The master manipulator refers to an electromechanical system with which the human operator interfaces. The master serves two purposes, one to interpret the operator’s position and transmit this information electronically to remote manipulators. Second, it should haptically display reaction forces that are generated at the environment. The other half of the kinesthetic coupling, the remote manipulator, is also an electromechanical system that performs the inverse task of the master except at the remote environment. Namely, the remote manipulator displays position commands received from the master and interprets forces generated by the environment for transmission back to the master, and hence the human operator. The problem of non-intuitive directional motion of the tool tip caused by the fulcrum effect can be resolved by matching the surgeon’s input motions to the end-effector of the instrument instead of the instrument handle. Hence, reversal of direction and unwanted scaling artefacts are removed. Since the remote center is placed at body-entry point local tissue stress can be minimized (The principle of “remote center kinematics” is explained in the Appendix). Electromechanical designed master and remote manipulators are not constrained to be kinematically identical. Therefore, additional degrees of freedom can be designed into computer controlled endoscopic instruments. As a result, an anthropomorphic master can intuitively control these degrees of freedom as long as Cartesian directions of position and orientation between the master and remote manipulator are preserved.
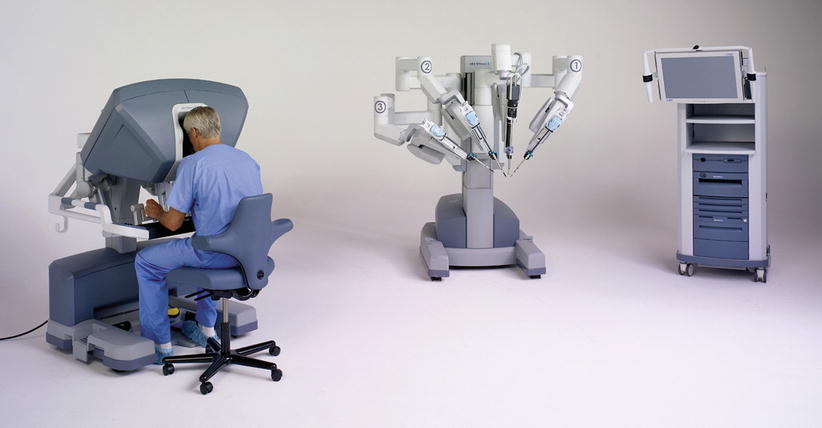
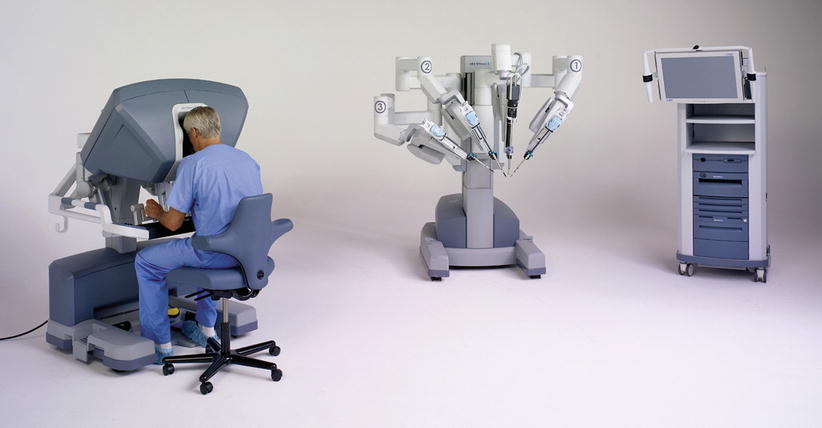
Fig. 1.5
The Da Vinci System™ is an example of state of the art surgical telemanipulation (Copyright Intuitive Surgical, Inc., used with permission)
Kinesthetic coupling is of limited use if the human cannot observe the results of his or her actions on the environment. The third key component of a tele-manipulator system is visual feedback. Together both haptic and visual feedbacks enable a human operator to perform useful work on a remote environment, while immersed in a reciprocal local virtual environment. Hand-eye coordination is restored to the surgeon as the system matches orientation and position of the remote manipulator instruments, as well as the surgeon’s hands on the master side in the same visual frame provided by the endoscope and the master side monitors. Appropriate transform algorithms can be used to convert the surgeon’s hand-eye coordinated motions on the master side to equivalent instrument motions on the slave side with respect to the endoscope.
Intuitive Surgical Da Vinci Tele-manipulation System
By far the most successful tele-manipulator in surgery to date is the da Vinci Surgical System (Intuitive Surgical Inc. Sunnyvale, CA), a 7 DOF telemanipulation system (6 DOF plus instrument function) (Fig. 1.5). The surgeon’s console houses both master manipulators and the video-display system. The master handles are serial link manipulators that act both as high resolution input devices, reading the position, orientation, and grip commands from the surgeon, and has haptic displays, transmitting forces and torques to the surgeon in response to various measured and synthetic force cues (bi-directional). The masters are gravity-compensated to minimize fatigue of the operator. The surgical site image is displayed for the surgeon through a high-resolution HDTV stereo display. This system projects the surgical site image atop the surgeon’s hands (via mirrored overlay optics), while the controller transforms the spatial motion of the instruments into the reference camera frame. Hereby, the system restores natural hand-eye coordination. Motion scaling allows for various ratios for master and manipulator motions and compensates for magnification. By activating a foot switch the operator is able to temporarily uncouple and reposition (clutch) the masters in the working field, while the instrument tips remain stationary enabling an optimal ergonomic working position. Targeted motions are usually slower (rarely exceed 1 Hz) than intrinsic hand tremor (4–8 Hz). By limiting motion command transmissions to lower frequencies, the system not only reduces its bandwidth requirements but also provides effective tremor filtration.
The stereoscope includes dual 3-chip cameras (two separate optical channels L/R) and is also controlled by the master handles. By activating a foot switch, the masters control stereoscope motion in four axes (rotational, insertion, two translational). A variety of stereoscopes is available (0° and 30° optics). By selecting the desired scope at the console, the masters are realigned automatically, according to the chosen viewing angle. The multi-image stereoscopic viewer can be formatted to display other on demand image inputs (e.g. HR monitoring, ultrasound). 3D imaging has been shown to improve endoscopic performance markedly, and HDTV resolution provides excellent detail of very small structures [18].
The patient side cart consists of three instrument manipulators and a camera manipulator. The camera manipulator has 4° of freedom and holds the stereoscopic endoscope. An interactive video display helps communication between the surgeon at the console and the patient side assistant. Multiple use end-effectors attach interchangeably to the three instrument manipulators, which feature an automated instrument recognition system (the integrated chip is programmed to set the correct instrument parameters and allow safe limited use only). The manipulator provides 3° of freedom (insertion and translation in two planes) while the end-effectors add another 3° of freedom (rotation and two additional pitch and yaw axes at the tip that also make up the grip motion). Therefore, a total of 6° of freedom are provided, allowing free motion and orientation of the instrument tip in space. The EndoWrist can be articulated 180° spherical and rotation up to 540° is possible. Instrument diameters are 5 or 8 mm. To minimize shear stress at the site of insertion, all four patient-side manipulators use remote-center technology. If the remote center is placed correctly within the body-entry port, the highest precision and lowest friction are provided. Switching between patient side manipulators, using one instrument to retract or hold tissue while operating with the two other arms, effectively allows for what is best described as “serial auto-assistance”. The variety of instruments has grown substantially over the past decade. More than 40 different tools for tissue handling and manipulation are available and now include a Vessel Sealer and a Linear Stapler. The da Vinci also allows for coupling of multiple consoles to facilitate teaching, including telestration and console pointers for proctoring of surgeon learners. Moreover, internet-based tele-presence teaching is now feasible. A skill simulator backpack can be connected to the da Vinci surgeon console allowing for exercising robotic surgery skills in a virtual 3D environment on the device itself. The latest generation of the da Vinci enables fluorescence imaging with Indocyanine Green [ICG] for real-time, image-guidance using near-infrared technology. ICG emits an infrared signal when excited by laser light in situ, which can be detected by the da Vinci computer core and displayed as a colorized overlay in the surgeons console. This allows target vessel detection, anastomotic patency assessment and evaluation of distal coronary flow during coronary bypass grafting. Other ongoing developments revolve around a robotic measurement method which combines the telemanipulation of a robotic instrument with stereoscopic image processing to measure and virtually display cardiac anatomy in 3D [19]. This would allow intraoperative measurement of mitral valve anatomy as well as relates to graft length, coronary size, etc.
DLR Telemanipulation System
The lightweight DLR MIRO (DLR Robotics and Mechatronics Center, Munich, Germany), weighs less than 10 kg, has seven joints, a kinematic length of 760 mm, and torque sensors in all joints that are designed for a broad range of surgical applications. Compliant control algorithms allow safe surgeon-patient interaction. The robot is designed in a modular way so that multiple arms can be linked and applied through the same interface. In its current preclinical version bimanual force feedback is provided using a standard haptic device (Omega7). Stereovision is provided using auto-stereoscopic display manipulation with forces and torque levels displayed at the master unit. The system is designed to allow remote surgical operation using classical surgical end effectors such as forceps and needle holders. The same arm can also be used to run a drilling tool. The tips of the DLR micro forceps have integrated sensors that provide haptic feedback. The system also features software to optimize port location and help with surgical planning (Fig. 1.6). To date, this system has not been designed to fulfill criteria for cardiac surgical applications and has not been applied clinically [20].
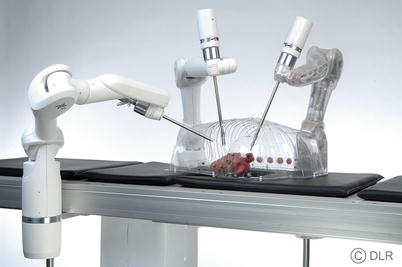
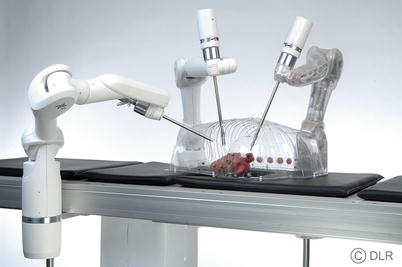
Fig. 1.6
The German Aerospace Center modular telemanipulator (Copyright German Aerospace Center; used with permission)
Hansen Medical Tele-manipulation Systems
Another example of a Telemanipulator is the Hansen Medical Sensei robot. This system is used to navigate a steerable sheath remotely for catheter guidance. The system accommodates catheters (i.e. for ablation) through the lumen of up to 8.5 Fr. Accurate catheter control is possible in three dimensions with 270° deflection in any direction. Motion is scalable up to a 1:4 ratio. The system is designed to replicate physician hand movements at the catheter tip. It provides real-time measurement and display of the proximal force along the shaft of the percutaneous catheter. Sensors measure small variations in force and display them in a visual format. Thus far, the system has been used most widely for electrophysiological applications, including catheter-based atrial fibrillation ablation. The use of robotic navigation for pulmonary vein isolation has been shown to be effective and reduces fluoroscopy time and operator radiation exposure [21]. Hansen recently introduced the Magellan Robotic System for peripheral vascular interventions in the cath lab.
Tele-manipulation in Cardiac Surgery
While tele-manipulation technology helps to restore the dexterity and precision of a distant operator (surgeon) working in a confined space, the choreography and flow of the surgical procedure still is different from open cardiac surgery. Port placement is paramount in reducing the degree of difficulty and ensuring the success of the procedure. Knowledge about the range of motion and kinetics of the remote manipulators is essential. The assessment of potential interferences between arms and the patient’s body is important to determine the optimal placement of ports. With regards to endoscopic bypass grafting, a number of different trajectories are required to perform both the proximal to distal internal thoracic artery dissection, the pericardiotomy, and final anastomosis. The theoretical ideal port triangle for each of these tasks varies accordingly. Further limitations are anatomical as the rigid rib cage prevents unlimited expansion (unlike in the abdomen) and limits potential access to all intercostal spaces. In order to gain room for intrathoracic instruments, the heart can be displaced downward from its close proximity to the sternum by CO2 insufflation. However, the tolerable intra-thoracic pressure with concomitant organ shifting is limited by the patient’s hemodynamics. Although insufflation pressures of up to 10 mmHg are well tolerated usually, this manoeuvre will result inevitably in elevated right ventricular filling pressure, which ultimately decreases cardiac output.
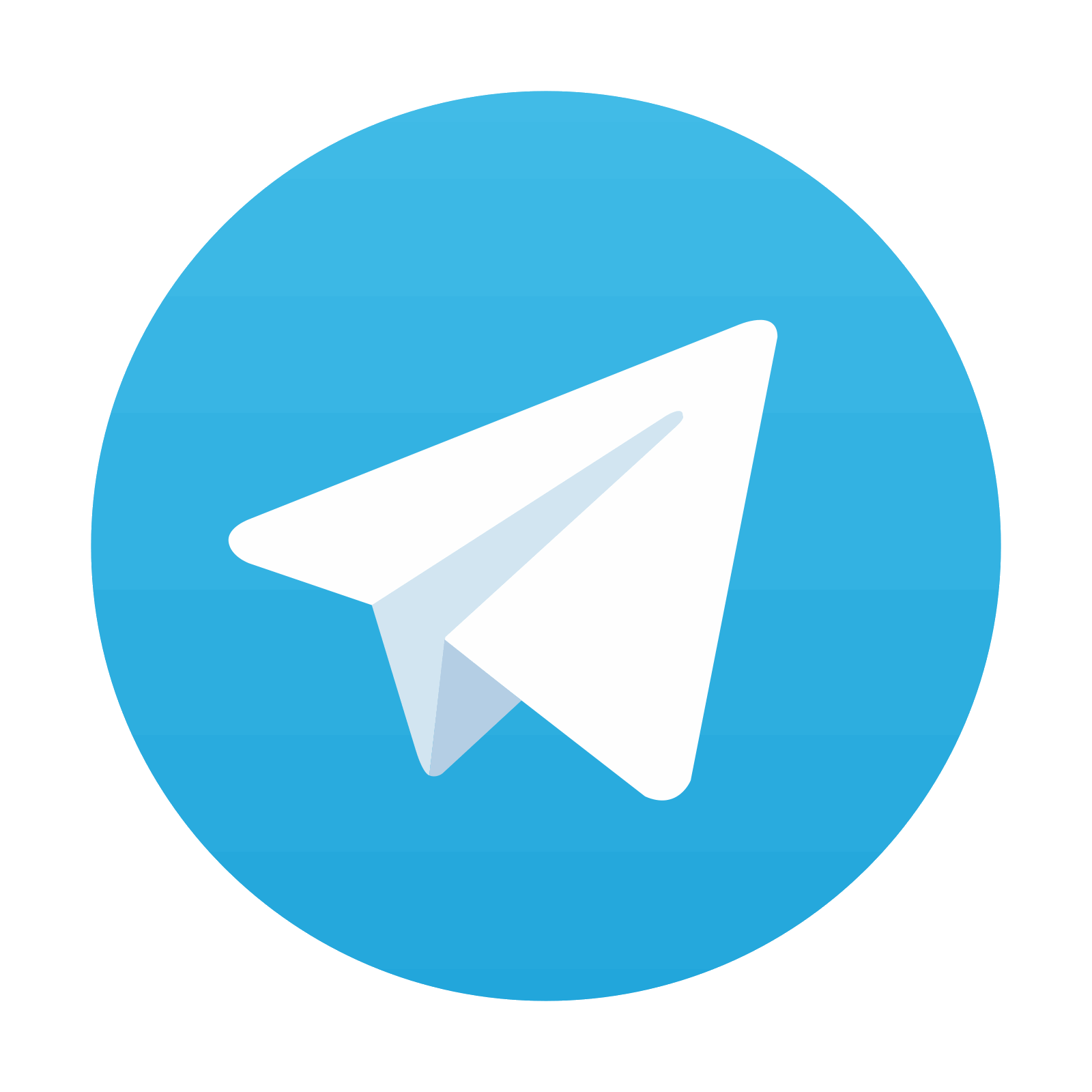
Stay updated, free articles. Join our Telegram channel
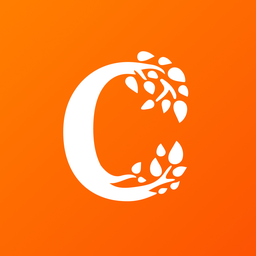
Full access? Get Clinical Tree
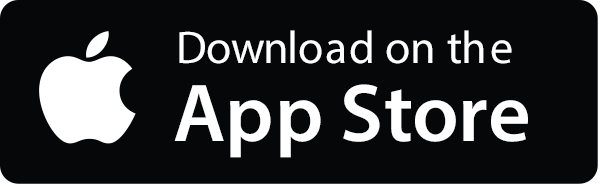
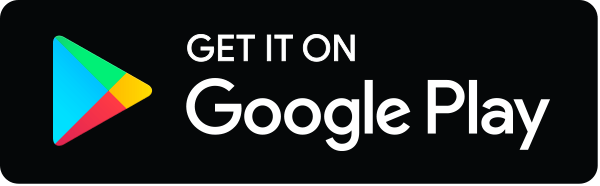