Background
Our objective was to explore the right ventricular (RV) dimensions and function and the effect of long-term intensive training in a large population of top level athletes using real-time three-dimensional (3D) echocardiography.
Methods
A total of 430 top-level athletes (220 endurance-trained athletes [ETAs] and 210 strength-trained athletes; 265 men [61.6%]; mean age 27.4 ± 10.1 years, range 18–40) and 250 healthy controls underwent a transthoracic echocardiographic examination. Three-dimensional RV measurements included serial short-axis reconstructions of the RV volumetric data sets, and the RV endocardial contour was traced with cross-reference to the long-axis images for identification of the tricuspid annulus. The end-diastolic and end-systolic RV volumes and ejection fraction were calculated off-line using the method of the summation of discs.
Results
The strength-trained athletes had a greater heart rate, body surface area, and systolic blood pressure at rest than the ETAs and controls. All RV diameters and 3D volumes and all transmitral and transtricuspid Doppler indexes were greater in the ETAs. Also, the left ventricular stroke volume, cardiac index, and pulmonary artery systolic pressure were greater in the ETAs, and all two-dimensional and 3D RV systolic indexes were comparable. On univariate analysis, the 3D RV end-diastolic volume was significantly associated with advanced age, male gender, duration of training, endurance training, increased left ventricular stroke volume, and pulmonary artery systolic pressure. On multivariate analysis, in the overall study population, the type and duration of training ( P < .01), pulmonary artery systolic pressure ( P < .01), and left ventricular stroke volume ( P < .001) were the only independent predictors of RV end-diastolic volume.
Conclusions
The results of the present study have delineated the upper limits of the RV dimensions in highly trained athletes as measured by real-time 3D echocardiography. The RV end-diastolic volume was significantly greater in the ETA than in the strength-trained athletes and controls.
Hemodynamic overload due to long-term training typically involves both left and right ventricles, inducing changes in the cardiac structure globally described as “athlete’s heart.” The cardiac adaptations can vary according to the type of sport. In particular, the isotonic exercise associated with endurance sports is responsible for a chronic volume overload, with a predominant increase in the left ventricular (LV) mass and end-diastolic diameters (eccentric hypertrophy). In contrast, isometric exercise, typical of strength disciplines, induces a prevalent increase in the LV mass and wall thickness (concentric hypertrophy).
A considerable body of echocardiographic studies has described the morphologic and functional adaptations of the left ventricle in athletes ; however, only a few studies have addressed the effect of long-term intensive training on right heart structure and function. The reasons for this imbalance appear, at least in part, to result from the complexity of the three-dimensional (3D) structure of the right ventricle and its dynamic characteristic of nonconcentric, but predominantly longitudinal, contraction, its retrosternal location, and the complex dynamic cooperation with the interventricular septum. A complex protocol of evaluation of the right ventricle using different echocardiographic sections in sequence was coded in detail by Foale et al.
Real-time 3D echocardiography overcomes the limitations of two-dimensional (2D) imaging methods. Also, the computation in the 3D space of the right ventricular (RV) volume and function throughout the cardiac cycle, without any geometric assumption, appears promising and more accurate than that available from 2D images.
The aim of the present study was to explore the RV dimensions and function using 3D echocardiography and the effect of long-term intensive training in a large population of competitive athletes.
Methods
Study Population
From January 2010 to October 2011, 440 top-level athletes were consecutively referred to the sports medicine outpatient clinic of Monaldi Hospital (Naples, Italy) for cardiovascular preparticipation screening and, afterward, to our echocardiographic laboratory for the purposes of the present study. We also studied 250 age- and gender-matched subjects without detectable cardiovascular disease and/or risk factors. The volunteer controls were all recruited from Naples (Italy), selected from our departments of cardiology among an initial cohort of 370 subjects investigated for work eligibility on the basis of their willingness to undergo an echocardiographic exam, and were examined in a single center (Monaldi Hospital, Naples, Italy). Of the initial 370 subjects investigated for work eligibility, 50 refused to be included in the echocardiographic protocol, and another 70 were excluded because of detectable risk factors. None of the selected control subjects included in the present study had cardiovascular structural or functional abnormalities or received any medication.
All subjects underwent a detailed history, physical examination, electrocardiography, ergometric test, transthoracic echocardiography, including Doppler studies, and real-time 3D RV echocardiography (RT3DE).
The exclusion criteria were coronary artery disease, arterial hypertension, valvular or congenital heart disease, bicuspid aortic valve, congestive heart failure, cardiomyopathy, diabetes mellitus, sinus tachycardia, the use of anabolic steroids or other illicit drugs, and inadequate echocardiographic image quality. In particular, no evidence of arrhythmogenic RV dysplasia signs was observed from the electrocardiographic and echocardiographic analysis (lack of prominent moderator band or saccular outpouching of the right ventricle) in any of the participants. Using these criteria, 10 subjects were excluded (3 for a bicuspid aortic valve, 1 for hypertrophic cardiomyopathy, 4 for the use of anabolic steroids, and 2 for inadequate echocardiographic image quality). Thus, our final study population consisted of 680 subjects.
The local ethics committee approved the study, and all subjects enrolled in the study gave informed consent.
On the basis of the training protocol and type of sports activity (mainly dynamic or static), the athletes were categorized into two groups: endurance-trained athletes (ETAs, n = 220) and strength-trained athletes (STAs, n = 210). Of the 430 athletes, 265 were men (61.6%), and the mean age was 27.4 ± 10.1 years (range 18-40; Table 1 ).
Characteristic | Controls (n = 250) | STAs (n = 210) | ETAs (n = 220) |
---|---|---|---|
Age (years) | 27.8 ± 10.3 | 28.5 ± 9.2 | 28.4 ± 11.1 |
Gender (n) | |||
Male | 145 | 140 | 145 |
Female | 105 | 70 | 75 |
HR (beats/min) | 72.5 ± 10.8 | 67.9 ± 9.8 | 51.1 ± 4.3 ∗ |
BSA (m 2 ) | 1.82 ± 0.6 | 1.89 ± 0.7 † | 1.83 ± 0.5 |
SBP (mm Hg) | 120.5 ± 6.83 | 133.4 ± 7.3 † | 115.8 ± 8.1 |
DBP (mm Hg) | 75.7 ± 4.4 | 78.5 ± 4.0 | 74.7 ± 4.9 |
∗ P < .001, ETAs vs STAs and controls.
Training Protocol
All the athletes were employed by teams in major professional sports associations and, therefore, were closely monitored during their training protocols by expert sports medicine specialists. All athletes had trained intensively 15–20 hours/week for more than 4 years. The ETA group (practicing long- and middle-distance swimming or running, soccer, or basketball) underwent intensive aerobic isotonic dynamic exercise at incremental workloads of 70–90% of their maximal heart rate. In particular, they performed 3 hours/day of incremental long-distance swimming (7,000 m/day divided into series of 400–800 m) or 3 hours/day of long-distance running and only 2 hours/week of weight lifting at a low workload. In contrast, the STA group (practicing weight lifting, martial arts, windsurfing) underwent anaerobic isometric static exercise at incremental workloads of 40–60% of their maximal heart rate. Their training protocol included 3 hours/week of short-distance running and 3 hours/day of weight lifting at a high workload.
Imaging Protocol
Standardized transthoracic echocardiography and Doppler examinations were performed with commercially available equipment in all subjects (Vivid E9, GE Healthcare, Milwaukee, WI). All the echocardiographic studies were performed during the early morning, before the training sessions. The specific views included the parasternal long- and short-axis views (at the mitral valve and papillary muscle level), apical four-, two-, and three-chamber views, and subcostal views, including respiratory motion of the inferior vena cava. Pulsed and continuous wave Doppler interrogation was performed on all four cardiac valves. All studies were reviewed and analyzed off-line by 2 independent observers who were unaware of the clinical characteristics of the study population. Specific measurements were made by the average of three to five cardiac cycles.
M- and B-mode Measurements
The LV mass was calculated using the Penn convention and indexed for height 2.7 (Cornell adjustment). The circumferential end-systolic stress was calculated as a measurement of LV afterload using a cylindrical model according to the following formula : ESSc (g/cm 2 ) = SBP × ½ Ds 2 {1 + [(½ Ds + ½ Ps) 2 /(½ Ds + ½ Ps) 2 ]}/(½ Ds + Ps) 2 − ½ Ds 2 , where ESSc is the circumferential end-systolic stress, SBP is the systolic blood pressure, Ds is the end-systolic diameter, and Ps is the posterior wall thickness in systole.
The LV ejection fraction was calculated using biplane Simpson’s rule from the apical four- and two-chamber views. The left atrial maximal volume was measured at the point of mitral valve opening using the biplane area-length method, and corrected for body surface area (BSA).
The RV end-diastolic chamber size was accurately assessed according to the recent American Society of Echocardiography guidelines for the echocardiographic assessment of the right heart in adults. In particular, three parameters included the measurement of the basal, midcavity, and longitudinal diameters from the apical four-chamber view at end-diastole ( Figure 1 ). The echocardiographic transducer was adjusted to the level of the RV chamber, with the goal of optimizing the RV chamber size.

The RV wall thickness was measured in diastole, preferably from the subcostal view, using either M-mode or 2D imaging.
The tricuspid annular plane systolic excursion was calculated as the index of RV longitudinal systolic function by placing an M-mode cursor through the tricuspid annulus in a standard apical four-chamber window and measuring the difference between the end-diastolic and end-systolic amount of longitudinal motion of the annulus (in millimeters). The RV end-systolic and end-diastolic cavity areas were traced in the apical four-chamber view, and the percentage of change in area was calculated.
Color Doppler Analysis
Valvular regurgitation was quantified from color Doppler imaging and categorized as absent, minimal (within normal limits), mild, moderate, or severe on the basis of the width of the vena contracta. Intermediate vena contracta values (3–7 mm) were confirmed using the proximal isovelocity surface area method.
Doppler-derived LV diastolic inflow was recorded in the apical four-chamber view by placing the sample volume at the tip level. Early (E′) diastolic tissue Doppler velocities were measured at the septal and lateral corners of the mitral annulus, and the mean between the two values was calculated. The LV stroke volume was calculated as the product of the LV outflow tract area and outflow tract time-velocity integral.
Pulsed Doppler evaluation of the RV diastolic indexes was performed in the apical four-chamber view by placing the sample volume at the tips of the tricuspid valve. The following measurements of global RV filling were obtained: E and A peak velocities (in m/sec), E/A ratio, and E-wave deceleration time.
To obtain a measure of RV myocardial function by tissue Doppler, the RV peak systolic velocity was assessed from the apical four-chamber view by placing the sample volume at the tricuspid annulus. Because this technique uses Doppler, special care is required to ensure optimal image orientation and avoid underestimation of the velocities.
The peak tricuspid regurgitant velocity was measured from the spectral profile of the tricuspid regurgitant jet in the parasternal long-axis view of the RV inflow tract, parasternal short-axis view, or apical four-chamber view. The greatest transvalvular velocity was used for calculation of the RV systolic pressure. The pulmonary artery systolic pressure (PASP) was then calculated by adding the right atrial pressure as estimated by the inferior vena cava respiratory index to the systolic transtricuspid gradient: PASP = 4V 2 + right atrial pressure, where V is the maximal velocity of the tricuspid regurgitant jet.
The PASP was assumed to equate to RV systolic pressure in the absence of pulmonic stenosis and/or RV outflow tract obstruction.
Real-time 3D RV Echocardiography
Three-dimensional acquisitions were obtained using the Vivid E9 scanner (Vivid E9, GE Healthcare, Milwaukee, WI) equipped with a central X4 transducer (frequency 3–4 MHz, volumetric frame rate 16–24 frame/sec, imaging depth 6–16 cm, rotation speed 6 Hz, and pulse length 2.5 cycles). Images were acquired from the apical four-chamber view with the patient in the left decubitus position during a breath hold of 5 seconds. Care was taken to include the entire LV volume within the pyramid-shaped 3D scan volume and to obtain a modified apical four-chamber view with focus on the right ventricle for RV analysis. In addition, the depth and width were adjusted to include the RV apex. The images were then transferred to an off-line workstation (4D Analysis, TomTec, Munich, Germany). Specially designed software was used for quantification of global RV and LV function. In particular, serial short-axis reconstructions of the RV volumetric data sets were obtained, and the RV endocardial contour was traced at 7-mm intervals with cross-reference to the long-axis images for identification of the tricuspid annulus. This protocol is identical to the one used in cardiac magnetic resonance imaging. The end-diastolic phase was defined as the peak of the R-wave of the QRS complex and the end-systolic phase as the first frame before the opening of the tricuspid valve. The papillary muscles and trabeculations were excluded from endocardial mapping. The end-diastolic and end-systolic RV volumes and ejection fraction were calculated off line using the method of summation of discs and semiautomated border detection. Valve opening and closure were identified for accurate volume determination. Using the same full-volume 3D echocardiographic data set, the epicardial boundaries of the RV wall were identified and traced to calculate an epicardial cast of the right ventricle at end-diastole. The volume of this cast was then subtracted from the endocardial cast and the volume of the RV myocardium derived.
Exercise Stress Test
All the athletes underwent an exercise stress test by bicycle ergometry in the upright position. The protocol involved increases at incremental workloads of 25 W every 2 minutes. The test was terminated if the athlete was unable to maintain a cycling frequency greater than 40 rpm or if maximal heart rate was achieved. The following functional indexes were assessed at peak effort: maximal heart rate, maximal systolic blood pressure, maximal workload (number of Watts achieved with the bicycle test), and the rate-pressure product (maximal heart rate × maximal systolic blood pressure).
Statistical Analysis
All the analyses were performed using SPSS, version 16.0, for Windows (SPSS, Chicago, IL). The data are presented as the mean ± SD. Analysis of variance and Newman-Keuls post hoc test for multiple comparisons were used to estimate the differences among the groups. Linear regression analysis and the partial correlation test by Pearson’s method were done to assess the univariate relations. On the basis of the theoretical and empirical limitations of ratio scaling, we checked whether this scaling approach removed the influence of body size using a simple bivariate correlation approach. Where ratio scaling failed to remove the influence of body size, we subsequently investigated the nature of the allometric relationships between the BSA and 3D RV volumes and determined whether allometric scaling for the BSA provided size-independent RV dimensional indexes. Allometric scaling of the general form y = a * x b was used.
To identify significant independent determinants of the absolute 3D RV end-diastolic volume in athletes, its individual association with clinical relevant and echocardiographic variables was assessed by multiple multivariate linear regression analysis. The following variables were included in the analysis: clinical data (age, gender, BSA, blood pressure, and type and duration of sports training) and standard echocardiographic indexes (LV volumes, LV mass index, LV stroke volume, LV ejection fraction, Doppler transmitral and transtricuspid inflow measurements, and PASP). These variables were selected according to their clinical relevance and potential effect on the right heart dimensions, as shown by previous reports. Variable selection was performed using multivariate linear regression analysis as an interactive stepwise backward elimination method, each time excluding the one variable with the greatest P value according to the Wald statistics. To minimize type 1 error rate inflation owing to multiple testing, statistical significance was defined as two-sided P < .01.
The reproducibility of RV 3D echocardiographic measurements was determined in all subjects. Intraobserver variability was determined using both Pearson’s bivariate two-tailed correlations and Bland-Altman analysis. Correlation coefficients, 95% CIs, and percentage of errors are reported.
Results
The clinical characteristics of the study population are listed in Table 1 . The mean age was comparable among the three groups. In accordance with the effects of the different training protocols, the STAs had a greater heart rate, BSA, and systolic blood pressure at rest than the ETAs and controls.
The STAs showed an increased sum of wall thickness (septum plus LV posterior wall), LV relative wall thickness, and circumferential end-systolic stress. In contrast, the LA volume and LV end-diastolic volume were greater in the ETAs ( Table 2 ).
Controls | STAs | ETAs | |
---|---|---|---|
Septal wall thickness (mm) | 8.7 ± 1.1 | 11.4 ± 2.4 ∗ | 9.6 ± 3.1 |
Posterior wall thickness (mm) | 8.4 ± 2.1 | 11.7 ± 1.6 ∗ | 9.3 ± 2.1 |
LV end-diastolic volume (mL) | 121.8 ± 13.7 | 126.2 ± 13.3 | 160.4 ± 13.6 † |
LV end-systolic volume (mL) | 37.1 ± 8.4 | 35.8 ± 8.3 | 40.7 ± 9.9 |
Relative diastolic wall thickness | 0.36 ± 0.04 | 0.44 ± 0.08 ∗ | 0.36 ± 0.06 |
LV mass index (g/m 2.7 ) | 48.6 ± 5.9 ‡ | 65.5 ± 8.7 | 63.5 ± 9.9 |
LV ESSc (g/cm 2 ) | 86.1 ± 10.2 | 159.6 ± 17.5 ∗ | 91.3 ± 15.2 |
LV ejection fraction (%) | 68.7 ± 4.3 | 67.5 ± 3.8 | 68.7 ± 4.7 |
Left atrial volume index (mL/m 2 ) | 24.8 ± 6.3 | 24.4± 8.4 | 29.4± 9.1 § |
∗ P < .01 STAs vs ETAs and controls.
† P < .001 ETAS vs STAs and controls.
‡ P < .0001 controls vs ETAs and STAs.
All RV diameters and all transmitral and transtricuspid Doppler indexes were greater in the ETAs, with an increased E peak velocity and E/A ratio. Also, the LV stroke volume and cardiac index were greater in the ETAs, and all RV systolic indexes were comparable ( Tables 3 and 4 ).
Variable | Controls | STAs | ETAs |
---|---|---|---|
RV wall thickness (mm) | 3.64± 1.2 (2.1–4.6) | 3.7 ± 1.1 (2.5–5) | 3.6 ± 0.9 (2.8–5.4) |
RV basal diameter (mm) | 32.5 ± 4.2 (26–39) | 33.3 ± 5.3 (27–40) | 38.7 ± 5.4 ∗ (33–45) |
RV middle ventricle diameter (mm) | 27.6 ± 4.4 (22–34) | 27.5 ± 6.4 (22–36) | 33.5 ± 5.4 ∗ (28–40) |
RV base-to-apex diameter (mm) | 76.6 ± 6.8 (68–84) | 77.8 ± 6.3 (68–86) | 80.5 ± 5.8 † (73–87) |
RV 3D end-diastolic volume (mL) | 126.3 ± 23.1 (110–155) | 140.3 ± 25.1 (120–165) | 171 ± 33.4 (138–210) ∗ |
RV 3D end-systolic volume (mL) | 56 ± 18.3 (36–78) | 62 ± 23.4 (38–87) | 66 ± 23.1 (40-93) |
Indexed RV 3D end-diastolic volume [mL/(m 2 ) 0.43 ] ‡ | 73.5 ± 13.1 (59–86) | 77.8 ± 11.3 (64–90) | 94.4 ± 20.4 (76–115) § |
Indexed RV 3D end-systolic volume [mL/(m 2 ) 0.32 ] ‡ | 33.4 ± 10.3 (19–44) | 35.8 ± 12.4 (21–47) | 37 ± 14.1 (23–51) |
RV sphericity index | 0.34 ± 0.08 (0.22–0.43) | 0.37 ± 0.07 (0.24–0.44) | 0.42 ± 0.07 † (0.33–0.5) |
TAPSE (mm) | 22.7 ± 2.2 (20–25) | 22.4 ± 2.4 (20–25) | 23.5 ± 2.8 (20–26) |
RV area change (%) | 49.6 ± 2.3 (46–51) | 48.7 ± 3.3 (47–52) | 49.5 ± 3.67 (47–53) |
RV 3D ejection fraction (%) | 58.7 ± 9.5 (46–66) | 59.8 ± 8.5 (47–68) | 63.5 ± 9.4 (48–68) |
∗ P < .001 ETAs vs STAs and controls.
† P < .01 ETAs vs STAS and controls.
‡ Allometric scaling (size independent).
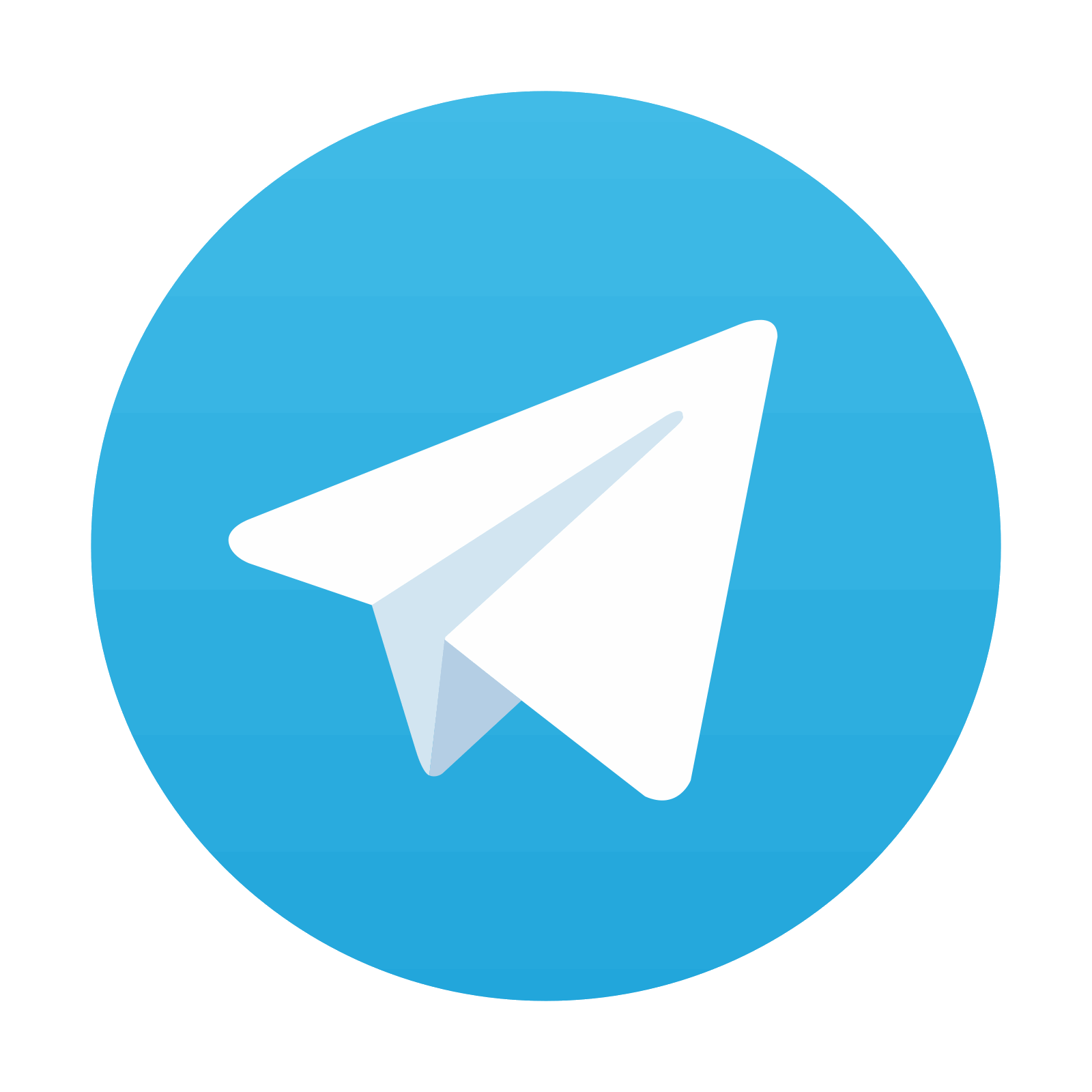
Stay updated, free articles. Join our Telegram channel
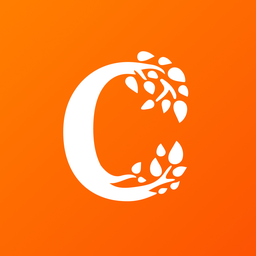
Full access? Get Clinical Tree
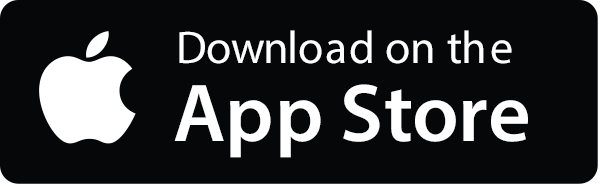
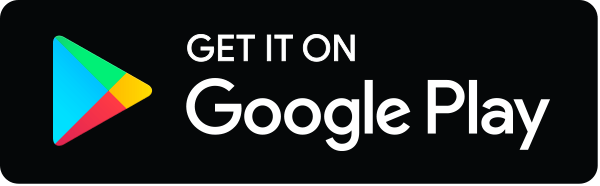