Background
There is little data on right ventricular (RV) remodeling patterns in complex congenital heart disease (CHD) patients with right ventricle to pulmonary artery (PA) conduits, and novel RV imaging modalities have not been explored in this population. Knowledge of the RV remodeling process is an important first step to future understanding and tracking of the RV response to pressure and volume overload in this diverse population. Three-dimensional knowledge-based reconstruction (3DKBR) derived from two-dimensional transthoracic echocardiography (TTE-3DKBR) is a novel approach to RV assessment. The aims of this study were twofold: (1) to assess the feasibility and accuracy of 3DKBR in patients with CHD with RV to PA conduits and (2) to characterize the three-dimensional shape of the RV across the spectrum of CHD with RV to PA conduits.
Methods
Seventeen patients with tetralogy of Fallot, pulmonary atresia with ventricular septal defect, or truncus arteriosus (mean age, 29 ± 8 years; 24% women) and a conduit referred for cardiac magnetic resonance imaging (CMR) were prospectively recruited and underwent TTE-3DKBR. TTE-3DKBR echocardiographic image acquisition was performed using a standard ultrasound scanner linked to a Ventripoint Medical Systems unit. The surface RV volumetric reconstruction was performed by transmitting two-dimensional data points to an online database and comparing these with a lesion-specific catalog to derive the RV reconstruction. Parameters analyzed were end-diastolic volume (EDV), end-systolic volume, and ejection fraction. Intertechnique agreement was assessed using Pearson’s correlation analysis, coefficients of variation, and Bland-Altman analysis. Three-dimensional shape comparisons of RV surface reconstructions were performed via automated validation testing of CMRs from 43 patients (mean age, 30 ± 8 years; 32% women) with RV to PA conduits (tetralogy of Fallot, n = 15; pulmonary atresia, n = 19; and truncus arteriosus, n = 9) distinct from patients in the 3DKBR comparison.
Results
There was good correlation and agreement between the two modalities: EDV, R = 0.77, P = .0004; end-systolic volume, R = 0.93, P < .0001; ejection fraction, R = 0.75, P < .0005. On Bland-Altman analyses, CMR EDV was slightly larger TTE-3DKBR, while EF was slightly higher by 3DKBR. Qualitative and quantitative assessment both demonstrated RV shape diversity based on surface reconstructions.
Conclusion
This study demonstrates that TTE-3DKBR is an alternative technology that can be used to assess the RV in patients with complex CHD with a conduit. A novel method was used to compare RV shapes in this important population, and our results draw specific attention to the fact that the RV both within and outside diagnostic groups has very different unpredictable shapes and should not be treated equally. Our findings should set into motion future work focused on indices of RV shape and their impact on overall RV function and clinical outcomes, hence defining optimal timing of conduit revision, which at the current time is very unclear.
Surgical placement of a right ventricle (RV)–to–pulmonary artery (PA) conduit plays an important role in the repair of complex congenital heart disease (CHD), specifically in conotruncal abnormalities, in which there is abnormal development of the right ventricular outflow tract (RVOT). Insertion of a RV-PA conduit has improved pulmonary blood flow in defects such as pulmonary atresia with ventricular septal defect (VSD), tetralogy of Fallot (TOF) and allows for a biventricular repair in truncus arteriosus (TA). The critical limitation of RV-PA conduits is the high failure rate over time, leading to symptoms and progressive RV dilatation and dysfunction. RV assessment is thought to be important to timing of reintervention, although there are little data to support this notion or to define optimal timing of intervention based on RV parameters. Furthermore, the RV has been assumed to be the same in patients with conduits regardless of the underlying CHD diagnosis. Knowledge of the RV remodeling process is an important first step to future understanding and tracking the RV response to pressure and volume overload in this diverse population. This line of investigation has been pursued in patients with TOF without conduits. This work has led to many novel studies, including the investigation of surgical techniques that address RV shape abnormalities to optimize RV function post-surgery. The RV in the complex conduit population has not been studied to date.
Two-dimensional (2D) transthoracic echocardiography (TTE) is limited in providing a quantitative assessment of RV volumes or ejection fraction (EF), as a result, cardiac magnetic resonance imaging (CMR) has become the reference standard. Quantitative three-dimensional (3D) echocardiography has been evaluated as a more economical and facile alternative to CMR. However, several limitations have been observed, including poor endocardial definition and consistent underestimation of RV volumes related to severe enlargement and abnormal remodeling such that the RV extends to outside the transducer imaging volume. Generation of a 3D RV model from 2D TTE images is possible with 3D knowledge-based reconstruction (TTE-3DKBR). This method has been validated in vitro and against CMR in patients with TOF, transposition of the great arteries, and in pulmonary hypertension. Specific anatomic landmarks are identified, and the proprietary reconstruction algorithm uses these landmarks to fit specific regions of the RV to various hearts in a catalog of patients with similar pathology. The algorithm then generates a 3D model based on all the subregions.
The aims of this study were twofold: (1) to assess the feasibility and accuracy of TTE-3DKBR in CHD patients with RV to PA conduits and (2) to characterize the 3D shape of the RV across the spectrum of patients with CHD with RV-PA conduits.
Methods
Study Design and Population
This was a single-center, prospective, observational study. The study was approved by the institutional research ethics board. Written informed consent was obtained from all patients prior to study enrollment. All patients were ≥18 years of age, had an RV-PA conduit, and had an underlying diagnosis of TOF, pulmonary atresia with VSD, or TA. Patients who were scheduled to undergo CMR for a clinical indication were eligible for inclusion. Exclusion criteria were any contraindication to CMR, including pacemaker or defibrillator, inability to comply with breath-hold instructions, and claustrophobia. Patients with a surgical history of a Rastelli procedure were excluded as the RV-PA conduit 3DKBR catalog did not extend to include this group. Patients were not screened for 2D transthoracic image quality prior to enrollment. Just before or after the CMR, an echocardiographic study was performed, including image acquisition for TTE-3DKBR. A total of 17 patients were prospectively enrolled for this TTE-3DKBR comparison. To determine if RV shape among patients with RV-PA conduit varied by underlying diagnosis, 3D CMR shape comparisons were performed. CMR studies of patients from our program were used for this purpose, for a total of 43 studies from patients with a conduit (TOF, n = 15; pulmonary atresia with VSD, n = 19; and TA, n = 9). These were patients who had a CMR and were not clinically eligible for another CMR and hence were not eligible for enrollment into the TTE-3DKBR comparison as described above. Details pertaining to conduit type, implantation approach, and conduit location were obtained and recorded from operative notes.
3D Knowledge-Based Reconstruction Method
Image Acquisition
Two-dimensional TTE images were acquired with standard ultrasound equipment (iE33 system and S5 transducer; Philips Medical Imaging, Andover, MA). This was connected to a specialized console and used with a magnetic field generator, located underneath the patient bed (Ventripoint Diagnostics Ltd, Seattle, WA). A standard 2D probe was used, with the addition of the magnetic field localizing system (Ascension Technology Corporation, Andover, MA) to track the transducer movements and position relative to the magnetic field transmitter located under the examination bed. The position and orientation of the receiver and thus the plane of the 2D picture can be computed and placed within the volume created by the magnetic transmitter. Images were recorded from the parasternal long-axis, RV inflow and outflow views, parasternal short-axis at both the papillary muscle and apical level apical four-chamber and focused RV apical views, with a focus on endocardial definition of the RV. Additional views were performed to demonstrate the RV-PA conduit. Each image was obtained at end-expiration with the patient in a fixed position throughout the study. The ultrasound scanner is linked to a computer (Ventripoint Medical Systems [VMS]; Ventripoint, Inc, Seattle, WA) through the video output, and every clip is digitized at 30 frames/sec. The magnetic field data are entered at the same time, and 3D localizing data are linked in the computer online. All images were acquired by two sonographers (M.L. and L.J.) trained in equipment use and image analysis. The entire acquisition protocol takes approximately 10 min.
Image Reconstruction
The images and spatial information recorded are stored on the VMS computer, which has an Internet connection to the centralized database. Offline analysis is performed by placing anatomic points using the VMS software. RV end-diastolic volume (EDV), end-systolic volume (ESV), and EF were calculated. End-diastole was selected manually on an apical four-chamber view, by visual determination of the time point at which RV area was largest immediately at or prior to tricuspid valve closure. End-systole was selected as the smallest area on the same apical four-chamber view. The same end-diastole–to–end-systole interval was automatically applied to all other acquisitions. Points corresponding to several anatomic landmarks were placed on TTE images at end-diastole and end-systole. For full 3DKBR, a minimum of nine key points need to be identified on the 2D images for a reconstruction to be possible ( Figure 1 , Table 1 ). However, we placed many more points; on average, 23 points were placed for reconstruction of the RV volumes in this study. Better endocardial definition allows a greater number of points to be placed. No additional anatomic tracing or border detection is required. RV endocardial points were placed at the base of trabeculations to the endocardial surface.

Site | No. of points ∗ |
---|---|
RV endocardium | 2 |
RV septum | 1 |
Pulmonic annulus | 1 |
Conal septum | 1 |
Tricuspid annulus | 3 |
Basal bulge | 0 |
Apex | 1 |
RV septal edge | 0 |
Subtricuspid | 0 |
Once the anatomic points on the 2D TTE images have been marked and localized in the magnetic 3D space, the surface RV volumetric reconstruction is performed by transmitting the data to the online database. The catalog CMR database consists of fully traced RV volumes of patients with RV-PA conduits with a wide spectrum of RV volumes and function. The points identified on the 2D images and relative 3D location is matched to the catalog database, and the best fit is computed using a proprietary algorithm. Once the minimum number of points has been placed, the first reconstruction can be performed. The reconstructed RV volume is projected on the 2D data set ( Figure 2 A), and point placement can be adjusted and/or more points can be added to refine the RV reconstruction and improve border alignment ( Figure 2 B). On TTE images with clear border misalignment giving rise to a very abnormal shape, suggesting a shift in patient position, images recorded after the patient had moved were excluded if all essential points were already placed, or the study was no longer able to be used if patient movement was not detected at the time of acquisition. All TTE studies were analyzed by a single observer (M.W.) with formal training in software use, who was blinded to CMR results. All analyses were performed offline after completion of acquisition. Five studies were performed for primary analysis before enrollment commenced. Analyses were also performed on all studies by two observers experienced with 3DKBR in assessment of interobserver variability.

CMR
Image Acquisition and Analysis
Cardiac magnetic resonance images were acquired using a 1.5-T scanner (GE Signa Twin Speed; GE Healthcare, Waukesha, WI). Retrospectively gated cine images were obtained with a steady-state free precession sequence (fast imaging employing steady-state acquisition). A stack of short-axis slices (1.3 msec TE; 3–4.5 msec TR; 45° Flip Angle; 10 mm thick; 0 interslice gap; 32–35 cm field of view; 224 × 224 matrix [phase, frequency]) from base to apex of the entire heart was acquired, from the tricuspid annulus to the level of the pulmonary valve. Short-axis cine slices were analyzed using volumetric analysis software (ReportCard 4; Neosoft LLC, New York, NY) to measure ventricular volumes and EFs using the Simpson method of disks using the standard technique of tracing the contours of the ventricular borders to the base of the endocardium, excluding trabeculations. The images with the largest and smallest ventricular volumes were selected as the end-diastolic and end-systolic images, respectively.
RV Shape Variation Assessment
To determine if RV shape among patients with RV-PA conduits varied by underlying diagnosis, 3D CMR shape comparisons were performed using the automated validation testing (AVT) algorithm. CMR studies of patients from our program were used for this purpose, for a total of 43 studies from patients with a conduit (TOF, n = 15; pulmonary atresia with VSD, n = 19; and TA, n = 9). None of these CMRs were from the 17 prospectively enrolled patients for 3DKBR comparison.
All shapes used in the quantitative AVT comparison were produced from CMR manual FullFit end-diastole tracings using VentriPoint’s piecewise smooth subdivision surface method. No 2D TTE images were utilized in this process. In order to compare shapes, the AVT program was fed (1) a test shape (i.e., one of the 43 CMR manual FullFit shapes) and (2) a hearts “catalog” for comparison (the remaining 42 CMR manual FullFit shapes). By analyzing which and how many of the 42 hearts the KBR used to reconstruct the test shape, the AVT program allows shape variability assessment. The AVT program samples several 3D anatomical points from the FullFit surface of the test shape. These points are inputted into the catalog of shapes and used to identify shapes with similar segmental or global shape, the combination of identified shapes is used to form a preliminary reconstruction. The resultant KBR reconstruction is quantitatively compared with the original FullFit phantom to find regions with discrepancies. To achieve the best possible match, the AVT then analyzes these discrepancies and adds additional points and incorporates additional corresponding shapes from the catalog, fine-tuning the reconstruction to more closely match the FullFit shape. This process is repeated until four reconstructions are ran in total, ensuring that the final fourth-round resultant VMS reconstruction is an almost perfect match to the test shape. This process was repeated for each of the 43 CMR studies included in this study.
The similarity between hearts was assessed qualitatively and by quantitative analyses. Shape comparisons were made from the CMR piecewise smooth subdivision surface FullFit images. It is important to note that all of the comparison hearts are scaled and normalized before being compared, so that two hearts with the exact same shape but different volume will match perfectly. Similarity is determined by how much any given “catalog” shape contributes to reconstruction of the “test” shape. This is a percentage that reflects how weighted the “catalog” shape is to provide the closest match to the “test” shape, as compared with other hearts in the “catalog.” In the case of “unique” hearts, many catalog shapes may be used to contribute to the reconstruction, none of which contribute a significant percentage to the final reconstruction. In the case of similar hearts, one or two hearts may contribute to the final reconstruction. A final check is performed to ensure that the test shape is truly unique by looking at the hearts contributing to the reconstruction, specifically to ensure that it is not that several similar heart shapes are contributing to an equally similar test shape. Another quantitative check is to look at maximum point distance discrepancies. This is done by orienting all hearts in the same plane as determined by the valve planes and apex. The meshes of the test shape and catalog hearts contributing to the single reconstruction are overlaid and compared. Differences in the distances orthogonal to each mesh surface at the reconstruction points are determined. A maximum mesh difference (maximum surface gap) of >1 cm implies that the shape reconstruction does not match the test shape well. Difference in volume calculated as percent volume difference (volume of test shape − volume of reconstruction/volume of test shape) was also determined. The volume difference is attributable to differences in shape given that the hearts are all scaled and normalized before the AVT algorithm is applied.
Statistical Analysis
Categorical variables were summarized using frequencies and percentages. Continuous variables were summarized using mean ± SD. The relationship between TTE-3DKBR- and CMR-derived RV volumes and EFs was evaluated using linear regression analysis with Pearson’s correlation coefficient. Bland-Altman analysis was performed to assess agreement between the two imaging modalities. Intraobserver (M.W.) agreement was assessed with repeated 3DKBR analysis 4 weeks after the initial analysis. Interobserver agreement was assessed for all study patients by two observers experienced with 3DKBR. Inter- and intraobserver agreement was quantified with intraclass correlation and coefficients of variation, the latter being the standard deviation of the difference of paired samples divided by the average of the paired samples. A P value < .05 has been considered statistically significant. Statistical analysis was performed using SPSS version 21.0 for Windows (SPSS, Chicago, IL).
Results
A total of 37 patients were approached, and 17 were enrolled for the TTE-3DKBR and CMR comparison ( Table 2 ). Eight patients were excluded due to CMR contraindications, eight patients had behavioral issues or claustrophobia and were/would be unable to tolerate CMR, and four patients did not consent to the study. The CMR RV EDV and ESV were 114 ± 32 and 76 ± 33 mL/m 2 , respectively. TTE-3DKBR volumes and EFs correlated well with CMR values: for EDV, R = 0.77; for ESV, R = 0.93; and for EF, R = 0.75 ( Figure 3 ). On Bland-Altman analyses, CMR EDV volumes were slightly larger than those obtained by TTE-3DKBR (ΔEDV CMR-TTE, 2.5 ± 19 mL; ΔESV CMR-TTE, 0.6 ± 10 mL), whereas EF tended to be slightly lower by CMR (ΔEF CMR-TTE, −2 ± 8%) ( Figure 3 ). Inter- and intraobserver analyses revealed relatively close agreement for volumes, with slightly larger variability for EF. For interobserver variability, intraclass correlation coefficients were 0.91 for EDV, 0.83 for ESV, and 0.80 for EF, and coefficients of variation were as follows: EDV, 8.8 ± 6.4%; ESV, 10.5 ± 8.3%; and EF, 16.2 ± 12.7%. For intraobserver analyses, intraclass correlation coefficients was 0.93 for EDV, 0.87 for ESV, and 0.80 for EF, and coefficients of variation were as follows: EDV, 7.4 ± 4.3%; ESV, 9.2 ± 7.4%; and EF, 12.3 ± 9.1%.
Characteristic | TTE-3DKBR comparison ( n = 17) | RV shape assessment ( n = 43) |
---|---|---|
Age (yrs) | 29 ± 8 | 30 ± 8 |
Women | 4 (24%) | 14 (32%) |
Underlying diagnosis | ||
TOF | 9 (53%) | 15 (35%) |
Pulmonary atresia with VSD | 6 (35%) | 19 (44%) |
TA | 2 (12%) | 9 (21%) |
BSA (m 2 ) | 1.8 ± 0.2 | 1.7 ± 0.3 |
Heart rate (beats/min) | 69 ± 14 | 73 ± 15 |
Conduit stenosis > mild | 13 (76%) | 36 (84%) |
Conduit regurgitation > mild | 6 (35%) | 17 (39%) |
Previous RVOT patch | 3 (18%) | 9 (21%) |
Tricuspid valve annuloplasty | 1 (6%) | 5 (12%) |

The RV shape assessment was performed with the CMR studies of 43 patients not included in the TTE-3DKBR comparison studies ( Table 2 ). The CMR-determined RV EDV, ESV, and EF were 212 ± 75 mL, 147 ± 73 mL, and 33 ± 10%, respectively. A large variation in RV shape was noted among this RV-PA conduit group. The three conduit subgroups could not be reliably discerned qualitatively looking at the 3D shapes. While all right RVs were dilated, a specific region of dilation could not be identified, with the exception of the apex. Specifically, the free wall, inferior, superior, and septal regions did not consistently show a shape pattern. TA hearts tended to have a smaller basal bulge, but it was not a reliable distinction, as we also found pulmonary atresia with VSD and TOF hearts with less pronounced basal bulge. Inflow and outflow annuli were in various orientations, and the conus region was not always dilated. Figure 4 illustrates the RV shape variability in this RV-PA conduit group. Quantitative assessment also demonstrated RV shape diversity, as several catalog hearts were needed to closely approximate any given test shape, as shown in Table 3 . These hearts were not similar, and all contributed a small percentage to the final reconstruction. Moreover, for any given diagnosis, the reconstruction used hearts with from all three diagnostic groups, indicating that there was minimal similarity within diagnostic groups. Eight hearts had a maximum surface gap > 1 cm (TOF, n = 4; pulmonary atresia with VSD, n = 2; TA, n = 2). Overall, the maximum surface gap was 8 ± 2 mm (TOF, 8.4 ± 3.2 mm; pulmonary atresia with VSD, 7.7 ± 2.1 mm; TA, 8.0 ± 2.5 mm; P = .72). The number of hearts used in the reconstruction was 7.1 ± 2.1 (range, 4–12). The number of hearts used in the reconstruction was similar regardless of the underlying diagnosis (TOF, 7.3 ± 2.0; pulmonary atresia with VSD, 7.1 ± 2.3; TA, 7.1 ± 1.8; P = .97). The number of hearts used in reconstructions that yielded a maximum surface gap ≥ 1 cm was significantly less than in those with a gap < 1 cm (5.5 ± 2.2 vs 7.5 ± 1.9, P = .01). This suggests that these eight hearts were particularly unique, such that few catalog hearts were similar in shape and hence able to be used in the reconstruction.

Test shape | Reconstruction composition for each test shape ∗ | Maximal surface gap (mm) | FullFit volume difference (%) |
---|---|---|---|
TOF | |||
TOF1 | PA1 21%, TOF2 21%, PA18 19%, TA5 12%, TOF14 9%, PA9 10%, TA2 8% | 6 | 1.9 |
TOF2 | TOF15 20%, TA9 19%, PA19 16%, TA8 12%, TOF14 11%, PA18 8%, PA1 7%, TOF5 4%, TA6 3% | 11.8 | 0.7 |
TOF3 | TOF4 35%, TOF5 29%, PA15 19%, PA4 17% | 7.8 | 8.6 |
TOF4 | TOF9 48%, TOF7 21%, TA3 12%, TOF10 10%, TOF3 9% | 12.8 | 33.2 |
TOF5 | TOF3 27%, TOF2 21%, PA12 13%, PA13 11%, TA8 10%, TOF15 10%, TOF6 9% | 8.9 | −2.8 |
TOF6 | TOF1 24%, TOF9 19%, TOF3 15%, TOF12 13%, PA15 9%, TOF4 7%, TOF3 6%, TOF8 3%, PA13 3%, PA7 1% | 7.5 | 5.4 |
TOF7 | TOF1 24%, TOF13 23%, TOF4 16%, PA13 14%, PA1 10%, TOF14 7%, TOF10 6% | 8.7 | 6.6 |
TOF8 | PA14 43%, PA12 23%, PA15 10%, TA6 8%, TOF15 7%, TA6 6%, PA11 3% | 2.7 | 0.1 |
TOF9 | TA7 37%, PA4 27%, TOF10 9%, TOF3 8%, PA13 6%, TOF6 3%, TOF12 3%, TOF7 4%, TOF4 3% | 6.2 | 0.1 |
TOF10 | PA7 24%, TOF4 15%, PA3 15%, PA17 14%, PA13 13%, TOF13 6%, PA11 4%, PA4 3%, TOF7 3%, TA1 3% | 8.4 | −10.8 |
TOF11 | TA1 39%, PA13 25%, TA8 13%, PA3 10%, TOF15 4%, PA1 4%, PA11 3%, PA3 1%, TOF13 1% | 4.5 | 1.8 |
TOF12 | PA5 37%, PA17 35%, TOF5 26%, PA6 2% | 14.8 | 9.4 |
TOF13 | TOF7 40%, TOF10 11%, TOF5 11%, TOF14 10%, PA11 10%, PA12 7%, PA3 5%, TA6 4%, PA13 2% | 6.9 | −5.5 |
TOF14 | TOF8 36%, TOF4 28%, TA6 17%, TOF10 10%, TOF3 9% | 11.2 | 26.3 |
TOF15 | PA12 39%, PA3 26%, PA5 11%, TOF6 8%, TA6 8%, TA8 7%, PA13 1% | 7.9 | 9.3 |
Pulmonary atresia with VSD | |||
PA1 | PA18 62%, TA8 16%, TOF14 11%, TA6 8%, PA19 3% | 7.9 | −1.9 |
PA2 | PA18 32%, TOF14 25%, PA19 21%, TOF12 12%, TA8 10% | 6.8 | −0.9 |
PA3 | TOF11 37%, TOF7 19%, PA13 19%, TOF3 15%, PA19 6%, PA5 2% | 7.6 | 7.5 |
PA4 | TOF8 31%, PA8 17%, TA9 15%, TOF9 15%, TA3 10%, TOF12 5%, PA17 4%, TOF13 3% | 9.1 | 12.1 |
PA5 | PA1 34%, TOF9 28%, TOF7 17%, TOF9 11%, PA1 8%, TOF3 4% | 7.9 | 4.1 |
PA6 | TA5 18%, PA13 18%, PA8 16%, PA19 15%, TOF12 11%, TOF14 8%, PA16 8%, TOF15 3%, TOF3 3% | 8.9 | −2.9 |
PA7 | TOF7 21%, TOF13 16%, TOF6 14%, PA17 12%, TOF3 8%, TOF4 7%, TA3 6%, PA5 5%, TA6 4%, PA4 4%, TOF9 3% | 5.3 | 4.3 |
PA8 | PA17 19%, PA6 18%, PA19 15%, PA4 15%, TA7 12%, PA18 10%, TOF8 4%, TA3 4%, PA9 3% | 6.4 | 4.8 |
PA9 | PA18 23%, PA12 20%, TOF2 17%, TA3 11%, TOF3 11%, TA6 6%, TOF8 6%, TA5 4%, PA18 2% | 8.8 | 5.5 |
PA10 | PA19 27%, PA5 20%, TOF15 14%, TOF3 11%, TA6 6%, PA9 5%, TA5 5%, PA14 4%, PA12 3%, PA1 2%, PA2 2%, TA5 1% | 4.2 | 2.2 |
PA11 | TOF15 53%, PA4 22%, PA8 16%, PA17 9% | 12.9 | −12.4 |
PA12 | TOF14 32%, TOF8 27%, PA1 16%, PA10 10%, TOF4 8%, TA4 7% | 7.7 | −7 |
PA13 | TOF7 29%, PA3 27%, TOF5 16%, PA16 14%, PA6 10%, TA1 4% | 7.4 | −2.2 |
PA14 | TOF8 54%, TOF13 15%, PA17 9%, TOF5 5%, TA8 5%, PA11 5%, TOF11 4%, TOF7 3% | 4.4 | −2.8 |
PA15 | PA19 43%, TOF3 15%, PA18 13%, PA8 12%, TA6 10%, PA14 7% | 7 | 7.8 |
PA16 | TOF15 28%, TOF7 13%, TOF9 12%, TOF5 10%, PA6 7%, PA13 7%, PA17 6%, PA19 6%, TA7 6%, TA1 5% | 7.7 | −0.7 |
PA17 | PA8 30%, TOF12 21%, PA13 20%, TOF10 15%, PA11 9%, TOF3 5% | 6.7 | 9.5 |
PA18 | PA16 30%, TOF6 28%, PA9 21%, TOF11 11%, TA4 10% | 7.3 | 0.9 |
PA19 | TOF8 48%, PA1 28%, PA6 15%, TOF3 9% | 11.8 | −14.6 |
TA | |||
TA1 | PA6 21%, PA16 18%, TOF11 18%, TA4 13%, TA5 13%, PA3 8%, TOF7 5%, PA12 4% | 7.4 | −3 |
TA2 | TOF5 32%, PA14 25%, TOF13 20%, TA7 13%, PA12 5%, PA16 5% | 5.8 | −8 |
TA3 | PA14 39%, PA5 22%, TOF4 20%, PA9 14%, TA4 5% | 6.1 | 2.4 |
TA4 | PA18 37%, PA16 16%, TOF11 11%, TA4 10%, TA8 9%, PA2 6%, PA12 5%, PA13 3%, TOF2 2% | 8.5 | 13.3 |
TA5 | TA7 33%, PA11 28%, TA6 14%,TOF15 8%,TA4 8%,PA9 5%,PA1 3% | 6.1 | −4.6 |
TA6 | TOF8 42%, PA1 28%, PA12 16%, TOF15 14% | 11.6 | 22.7 |
TA7 | TA5 42%, TA8 23%, PA14 10%, TA9 9%, PA16 5%, PA1 4%, TA6 4%, TA3 3% | 6.3 | 6.3 |
TA8 | PA1 31%, TA7 20%, TOF11 18%, PA14 13%, PA18 7%, TOF10 6%, TOF14 3%, PA9 2% | 7.9 | −4.8 |
TA9 | PA16 30%, TOF2 26%, TOF3 11%, TA8 10%, TOF5 8%, TOF12 6%, TOF15 5%, PA17 2%, TOF2 2% | 12.6 | 14.7 |
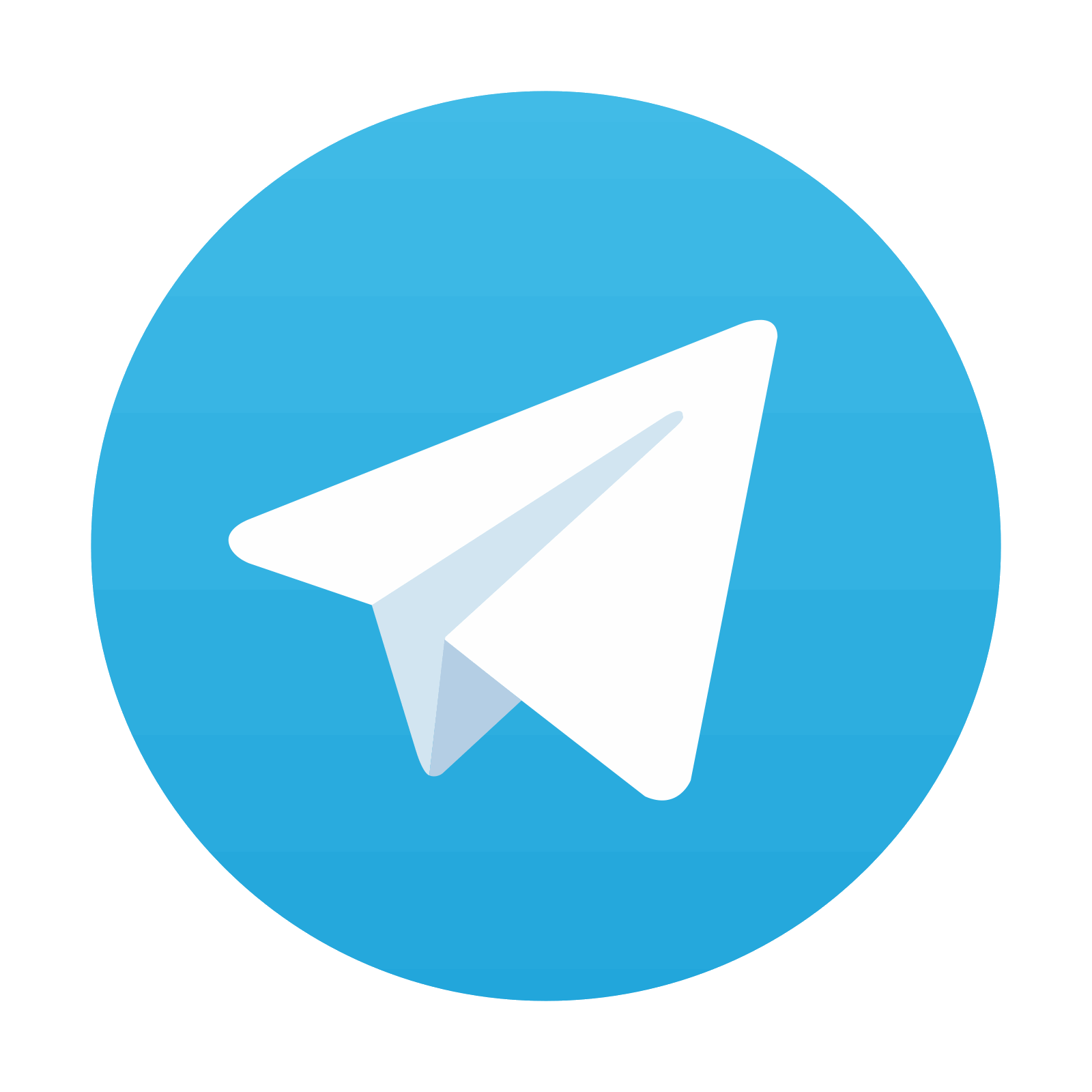
Stay updated, free articles. Join our Telegram channel
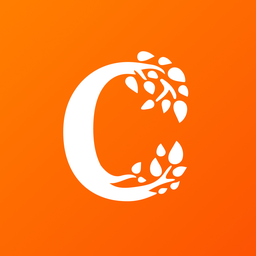
Full access? Get Clinical Tree
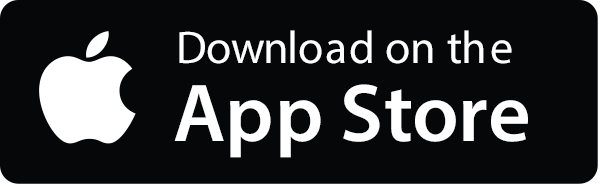
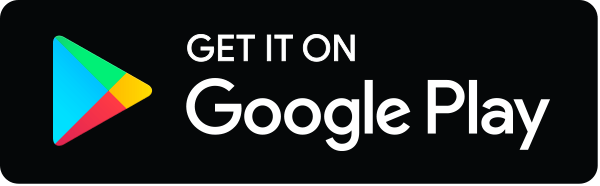
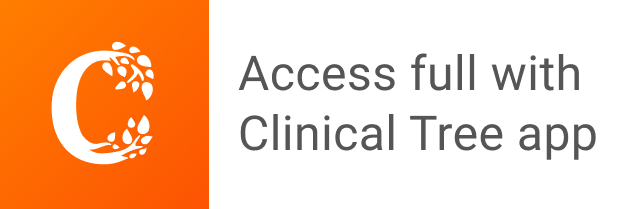