Introduction
For end-stage heart failure (HF), heart transplantation or implantation of a left ventricular assist device (LVAD) is lifesaving and provides a significant improvement in quality of life. Despite the therapeutic success of LVADs, concomitant right ventricular failure (RVF) and the lack of appropriate long-term, right ventricular (RV) support devices has limited the full potential that mechanical circulatory support (MCS) can offer to patients with biventricular failure.
Despite the relatively low rate of temporary RV assist device (RVAD) support in the United States, a significant number of patients who have been successfully discharged after receiving an LVAD remain symptomatic from RVF and undergo recurrent readmissions for the management of HF. In addition, delayed RVF is being increasingly recognized in a subset of patients on chronic LVAD support. In this chapter, we will discuss the pathophysiology of RVF, which can be potentially exacerbated after LVAD implantation, and the nonsurgical and surgical management strategies to address this extremely important issue.
Physiology and anatomy of the right ventricle
The RV is a complex three-dimensional structure that appears triangular in sagittal section, unlike the cone-shaped left ventricle (LV) chamber ( Fig. 14.1 ). Both the RV and LV are linked with a common meshwork of fibers that encircle the ventricles ( Fig. 14.2 ). The muscle mass of the RV is approximately one-sixth that of the LV and, under normal conditions, it functions in a low-pressured working environment.


Another uniqueness of RV structure is its high level of “reinforcement” with interweaving strands of muscle (trabeculae carneae) and a coarse endocardial inner surface. The pattern of RV trabeculae carneae in the ventricle is an efficient means of gaining power without excessively thickening the wall of the chamber (normally 3–5 mm in thickness). In patients with increased RV pressure, the trabeculae are more likely to be coarser, which may result in substantial alterations of RV volume, mass, and ejection fraction.
In addition, the shared interventricular septum (IVS) plays an important role in RV output. It has been suggested that a substantial portion of RV systolic pressure and stroke volume results from LV contraction, much of which is contributed through the IVS. As the IVS contracts, it adds to the contraction effort of the RV free wall and may in fact contribute as much as 40% to the output of the RV. In addition, the contracting free wall of the RV pushes against the IVS as its supporting structure. This ventricular interdependence of RV output on the IVS and on LV contractility has been demonstrated in animal models. As discussed later in the chapter, this has particular importance once an LVAD is implanted because it can significantly alter the loading conditions of both the RV and LV and their respective geometric relationship to each other.
Under normal baseline conditions, the unique anatomy, myocardial ultrastructure, and coronary blood flow physiology of the RV reflect the characteristics of a high-volume/low-pressure pump. Because of low pulmonary vascular resistance (PVR), the RV pumps the same stroke volume as the LV does, but with ~ 25% of the stroke work. Contraction of the extremely compliant, thin-walled RV (by virtue of the Laplace relationship) is a process starting at the inlet, then free wall, and subsequently ending at the infundibulum. Of note, unlike the LV, in which coronary perfusion is predominately during diastole, the low-pressured RV is perfused throughout the cardiac cycle. Hence, significant elevations in the RV end-diastolic pressure, without a concomitant increase in mean arterial pressure, may be accompanied by ischemia in a relatively hypertrophied RV. In chronic HF, the RV pressure-volume loop shifts from a normal trapezoidal shape, reflecting its high efficiency/low impedance, to a rectangular shape, which becomes indistinguishable from a normal LV pressure-volume loop, suggesting an ongoing RV adaptation to increased volume overload ( Fig. 14.3 ).

Right ventricular failure in patients with chronic heart failure after lvad
Definition of Right Ventricular Failure
The common working definition of RVF according to the Interagency Registry for Mechanically Assisted Circulatory Support (INTERMACS) is a persistence of signs and symptoms of RV dysfunction evident by central venous pressure (CVP) > 18 mm Hg with a cardiac index < 2.0 L/min/m 2 and in the absence of increased pulmonary capillary wedge pressure (PCWP) > 18 mm Hg, cardiac tamponade, ventricular arrhythmias, or pneumothorax. Alternatively, placement of an RVAD or the use of intravenous inotropes or inhaled nitric oxide for >14 days post-LVAD implantation fits into this definition. The development of RVF that requires RVAD support in LVAD recipients is associated with high mortality irrespective of the timing of device insertion Patients who require RVAD support have higher in-hospital mortality (48% vs. 9.5%) and worse 1-year survival rates (40% vs. 82%) compared to those who received LVAD only.
Pathophysiology of Right Ventricular Failure After LVAD Placement
The pathophysiologic mechanisms that lead to manifestations of RVF are multifactorial, with some related to preexisting conditions and others to the hemodynamic changes brought on by the device. First, in patients with generic “dilated” cardiomyopathies (whether genetic predisposition, chemotherapy induced, or other mechanisms), it is expected that the same cardiomyopathic processes that affect the LV will also involve the RV. To a large extent, the decrement in RV systolic and diastolic function and RV dilation is likely determined by this pathologic process and is further exacerbated by the afterload imposed by chronic pulmonary hypertension. Similarly, in patients with ischemic cardiomyopathy, coronary artery perfusion may be compromised by atherosclerotic disease in the distribution of the right coronary artery. The malperfusion can also be further exacerbated by decreased perfusion pressure related to high CVP and low systemic blood pressure, which are the defining characteristics of patients in end-stage HF.
Cardiopulmonary bypass during the surgery can also exacerbate RVF by causing an acute increase in PVR, likely due to inflammatory mediators. There is also a decrease in vasodilating mediators, such as nitric oxide and prostacyclin, with an increase in vasoconstricting mediators such as thromboxane A2 and endothelin. Blood transfusion and protamine administration have been shown to cause pulmonary vasoconstriction and exacerbate RV dysfunction as well. Many pulmonary vasodilators have been utilized to treat acute increases in PVR, including inhaled nitric oxide, prostaglandin E1 and prostacyclin, phosphodiesterase 5 inhibitors, and endothelin receptor antagonists. While these causes of postoperative pulmonary vasoconstriction have been well studied in the general cardiac surgery patient, there is a dearth of evidence analyzing the relative importance of these mechanisms specifically in the MCS population.
During the perioperative phase, the altered physiology created by the LVAD may also exacerbate RVF by several mechanisms. The effect of ventricular interdependence is most prominent in the setting of loading changes after LVAD implantation. Excessive LV unloading results in leftward shift of the IVS, thus jeopardizing efficient RV contraction and output. In addition, augmentation of cardiac output and systemic blood flow by the LVAD increases the amount of venous return to the failing RV. In the absence of a significant and immediate reduction in RV afterload, RV function may become compromised.
Right venticular afterload sensitivity and adaptation
LVAD placement causes dramatic changes in hemodynamic markers of RV load and function. One study examined hemodynamic changes in three phases: pre-LVAD, early post-LVAD (within 6 months of implantation), and prolonged LVAD therapy (19–36 months after implantation). In the early post-LVAD period, there were significant decreases in PCWP (25–10 mm Hg), effective arterial elastance (Ea; 1.04–0.43 mm Hg/mL), and PVR (2.22–1.50 Wood units), while pulmonary vascular compliance (PCA, 2.00–4.03 mL/mm Hg) increased significantly, confirming that LVAD placement reduces both resistive and pulsatile components of RV load. However, CVP did not decrease, and the CVP:PCWP ratio, an independent predictor of RVF post-LVAD, increased significantly (0.47–0.85). The data from this study suggest that despite the favorable impact of LVADs on reducing RV afterload in the early perioperative period, RV contractile dysfunction is the dominant determinant of RVF and is likely exacerbated by increased venous return. Hence, perioperative intervention continues to rely on the use of inotropes for contractile augmentation of the RV.
With prolonged LVAD therapy, PCWP and Ea remained significantly decreased (12 and 0.38 mm Hg/mL, respectively) from pre-LVAD values but were unchanged from the early post-LVAD values. In contrast, PVR further decreased between the early post-LVAD and prolonged LVAD treatment periods (1.50–1.17 Wood units). While CVP remained unchanged over both periods, CVP:PCWP decreased significantly from the early post-LVAD values (0.85–0.56) returning to baseline pre-LVAD values. These results suggest that continued LVAD support beyond the perioperative period decreases RV afterload and is coupled to improved RV contractile performance.
Preoperative evaluation and predictive risk scores
The importance of RVF predictors in the setting of LVAD implantation was recognized early, as evidenced by the numerous attempts to identify independent risk factors and develop RVF predictive scores. Since there are limited options for managing RVF, the purpose of these risk scores was to improve patient selection and outcomes by recognizing the need for biventricular assist devices (BIVADs) at the time of LVAD implantation. Existing risk prediction models incorporate a combination of demographics, markers of end-organ dysfunction, inotrope dependency, prior open chest procedures, and/or hemodynamic parameters. However, certain common limitations have hindered the clinical usefulness of these risk scores: poor consistency in the definition of RVF, heterogeneity of patient populations, and variability in the types of LVADs included in each study.
One recent report investigated the use of a Bayesian statistical model to provide more accurate predictions of acute, early, and late RVF based on preoperative variables. The accuracies of all three Bayesian models were 91%–97%, with a sensitivity of 90% and specificity of 98%–99%, significantly outperforming previously published risk scores. Bayesian models differ from standard multivariate analyses in that they consider the interrelationships and conditional probabilities among independent variables, making them particularly suited for combining a large sets of risk factors (each of the three independent models in this report consisted of 33–34 preoperative variables). These algorithms better reflect human logic in prioritizing dynamic clinical information but also benefit from the plethora of evidence provided by the INTERMACS.
Imaging techniques may also aid in preoperative risk assessment. Echocardiography in particular has shown promise in evaluating parameters of RV dysfunction. Tricuspid annular plane systolic excursion (TAPSE) < 7.5 mm was 91% specific and 46% sensitive in predicting post-LVAD RVF. Low TAPSE has not been established as a predictor of RV longitudinal function, likely because TAPSE is only a regional marker of RV function and assumes the motion of the RV free wall base represents the function of other segments. An RV:LV diameter ratio greater than 0.75 can be used as a surrogate for disproportionate RV remodeling and demonstrated an independent association with RVF. Some studies have shown that RVF after LVAD implantation is associated with decreased peak systolic longitudinal strain of the basal RV free wall (− 20.7% vs. − 14.9%) and RV global longitudinal strain below − 13%, while others found no association. The inconsistent results between different studies evaluating echocardiographic parameters may be partly explained by user variability.
One recent study evaluated noninvasively measured preoperative liver stiffness (LS) using transient elastography, which demonstrated that LS is a predictor of post-LVAD RVF and may also be used for optimization of RV function in the perioperative period. Chemokine receptor regulation patterns may also play a major role in prognostication, with one report showing that pre-LVAD chemokine receptor downregulation is associated with RVF and increased mortality after implantation.
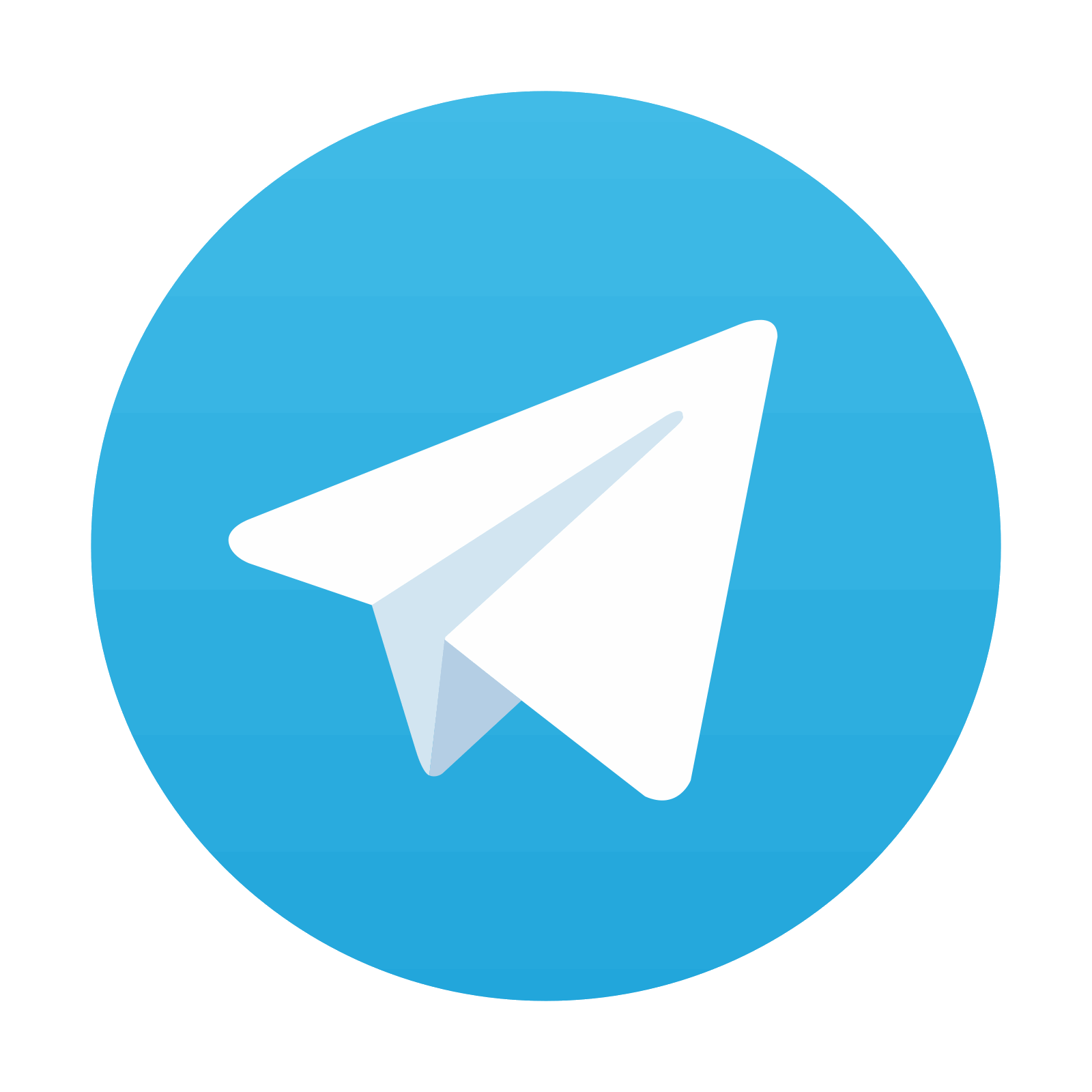
Stay updated, free articles. Join our Telegram channel
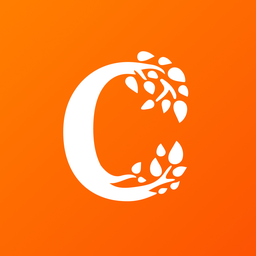
Full access? Get Clinical Tree
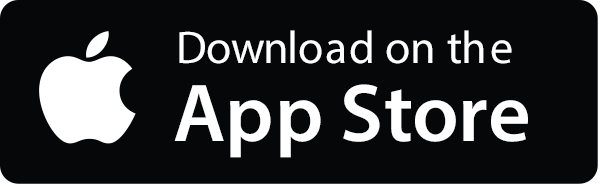
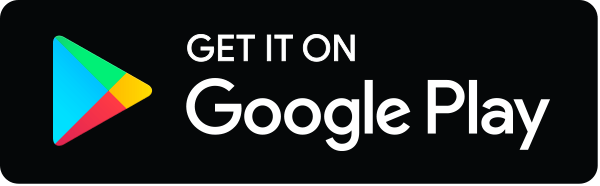