Fig. 18.1
Oxygen cascade
Considerable evidence suggests that exercise training programs may alleviate symptoms and improve long-term outcome in various diseases such as chronic obstructive pulmonary disease (COPD), diabetes, arterial hypertension, heart failure (HF), coronary artery disease, cancer, and neuropsychiatric disorders. Over the last 15 years, some clinicians have hypothesized that implementation of exercise training programs prior to and/or following surgery had the potential to “recondition” the patient by increasing aerobic fitness and thereby minimizing perioperative risk of major organ dysfunction while enhancing functional recovery soon after surgery.
In this chapter, we will review the current knowledge regarding physiological impairment observed in thoracic surgical patients, the mechanisms underlying endurance and strength exercise-induced improvement in physical fitness, and the efficacy of exercise training program when prescribed prior to and/or after surgery.
18.2 Physical Fitness in Thoracic Surgical Candidates
18.2.1 Assessment of Fitness Before Surgery
Cardiopulmonary exercise testing (CPET) on a cycloergometer or a treadmill represents the gold standard to assess patient’s physical fitness and the effectiveness of physical training interventions. Besides VO2peak (highest value attained during CPET) and VO2max (plateau level of VO2 achieved beyond which no increase in effort can raise it further), other parameters such as peak workload or peak power (Wmax), peak heart rate (peakHR), O2 pulse (VO2/HR), ventilator equivalent for CO2 (ratio of minute ventilation to the production of carbon dioxide, VE/VCO2), anaerobic threshold (AT), and respiratory gas exchange ratio (VCO2/VO2) all characterize the patient’s aerobic capacity. Overall, the predicted VO2max of any individual takes into account age, gender, height, and lean body mass. Alternate testing modalities have also been developed and validated to provide physiological surrogates of patient’s physical fitness as derived from stair climbing (speed of ascent, number of stairs) and the shuttle test or the 6-min walk test (6MWT, distance) (Table 18.1). In elderly and “frail” patients, simple tests of active mobilization such as the gait speed test (time needed to walk 5 m), maximal handgrip strength test (dynamometer), recording of all movement throughout the day (pedometer, accelerometer), the mini-mental test, and subjective performance scoring status (e.g., Karnofsky Performance Status) all complement valuable information on patient physical autonomy and bear important prognostic significance.
Table 18.1
Cardiorespiratory fitness testing modalities
Cardiopulmonary exercise testing CPET | Stair climbing or other physical stress tests | Shuttle or 6-min walk test | Age-predicted HR test | |
---|---|---|---|---|
Maximal | Submaximal | |||
Principle | Direct measurements of VO2, VCO2, HR, BP, and airflow | Estimated VO2max from highest workload, HR achieved | Distance (m) | Workload achieved at 70–85 % PredHR |
Equipment | Cycle ergometer/treadmill Expired–inspired gas HR (SpO2, BP) | Stair climbinga (6 floors) or cycle ergometer/treadmill (SpO2,BP, ECG) | 30-m corridor monitor HR, SpO2, stop watch | Cycle ergometer/treadmill monitor HR, SpO2, BP (ECG), stop watch |
Duration | 8–12 min | 5–20 min | 4–6 min | 5–20 min |
Operative risk | ||||
Low | VO2 max >20 ml/kg/min | >22 m altitude or 6 floors or 15 m/min | >600 m | |
Moderate | VO2 max 15–20 ml/kg/min | 8–20 m alt. or 3–5 floors | ||
High | VO2 max 10–15 ml/kg/min | 3–7 m alt. or 1–2 floors | 400 m | |
Very High | VO2 max < 10 ml/kg/min | <2.4 m alt. or 1 floor |
Guidelines issued from the American College of Chest Physicians (ACCP), from the British Thoracic Society (BTS), and jointly from the European Society of Thoracic Surgeons and the European Respiratory Society (ESTS/ERS) all recommend performing CPET whenever the diffusion capacity for carbon monoxide (DLCO) and/or forced expiratory volume in 1 s (FEV1) are below 80 % of predicted values [2–4]. The scientific rationale to perform CPET is to identify “unfit” subjects – those with low VO2peak – who might not be able to sustain the postoperative physiological impairments and the increased metabolic burden consequent to the surgical-induced neuroendocrine and inflammatory responses. Cutoff values of 15–16 ml.kg-1.min-1 VO2max (four METs) and 10–12 ml.kg-1.min-1 anaerobic threshold (three METs) have been shown to be helpful in discriminating patients at low–moderate risk and those at high (or very high) risk of major postoperative complications.
Importantly, patients with lung cancer awaiting surgery and particularly those receiving chemotherapeutic agents present VO2max on average 25–30 % lower than age- and gender-matched individuals (sedentary, active, or trained) (Fig. 18.2).


Fig. 18.2
Aerobic capacity assessed in healthy men and women (sedentary, active, and trained) and in patients with lung cancer receiving or not neoadjuvant chemotherapy
18.2.2 Causes of Poor Physical Fitness
Among thoracic surgical patients, the loss of muscular mass and aerobic physical fitness is often multifactorial as a result of aging, physical inactivity, tobacco-related illnesses, tumor burden, and chemotherapy, with no single organ or step of the oxygen cascade being identified as solely responsible.
Based on CPET, poor aerobic physical fitness is primarily linked to respiratory limitations (ventilator and gas exchange capacity), cardiovascular limitations (cardiac and vascular components, hemoglobin level), skeletal muscle limitations (muscular deconditioning, joint disorders, or neurological deficits), or a combination of these factors. The age-related decline in VO2max is largely attributed to impaired peripheral oxygen utilization coupled to the loss of lean body mass and decreased HR reserve (downregulation of β-adrenergic receptor and cardiac autonomic imbalance) that limits the exercise-induced increase in cardiac output. Likewise, a sedentary lifestyle, malnutrition, and prolonged immobilization have all been associated with low VO2max owing to lower heart rate response, loss of skeletal muscle mass, and impaired mitochondrial oxidative capacity.
18.2.3 Stress- and Inactivity-Induced Muscle Wasting
After surgery, afferent nerve signals from the injured tissues and pro-inflammatory cytokines released from activated leukocytes, fibroblasts, and endothelial cells all activate the sympathetic nervous system and the hypothalamic–pituitary axis. This so-called surgical stress-induced neuroendocrine and inflammatory response is proportional to the extent of tissue trauma. Concomitant to the peak release of inflammatory mediators and counter-regulatory hormones (cortisol, catecholamines, and glucagon), basal VO2 and VCO2 have been shown to increase by 10–25 % within the first 2 days after thoracic surgery, peaking at 30–45 % in patients with pneumonia [5, 6]. This hypermetabolic state reflects increased synthesis of acute phase proteins in the liver and enhanced tissue repair activity involving leukocytes, fibroblasts, and mesenchymal cells. The high levels of counter-regulatory hormones lead to a decrease in glucose cellular uptake/utilization (insulin resistance) and promote the breakdown of skeletal and visceral proteins into amino acids, as well as degradation of fat into glycerol and free fatty acids. Both amino acids and glycerol serve as substrates for hepatic neoglucogenesis and protein synthesis, while energy needs are predominantly met by free fatty acids in most tissues, except a few obligate glucose users (e.g., leukocytes, red blood cells, neurons).
Following major surgery, urinary nitrogen excretion increases to 40–100 g per day reflecting early muscle wasting (loss of 2–4 kg skeletal muscles) that takes several weeks for complete recovery [7]. The ensuing muscle weakness and fatigability when completing minor tasks impede early mobilization and return to functional autonomy. Frail subjects with sarcopenia and altered capacity to utilize nutrients are prone to experience postoperative multiorgan dysfunction resulting in admission to intensive care units (ICU), prolonged hospital stay, and discharge in institutional care facilities.
Besides the neuroendocrine and inflammatory components, physical inactivity associated with the intraoperative and postoperative period causes maladaptive changes in organ components of oxygen transport. Muscular disuse associated with short immobilization periods (5–10 days) has been shown to result in loss of muscle mass and strength due to an accelerated protein breakdown and in lower VO2max (−10–20 %) owing to reduced cardiac output and reduced red blood cell mass [8]. Interestingly, among all skeletal muscles, the diaphragm is most prone to inactivity-induced proteolysis leading to the so-called ventilation-induced diaphragmatic dysfunction. Unlike nonrespiratory muscles in the limbs or thoracoabdominal wall, the functioning and morphology of the diaphragm have been shown to be sensitive to ventilation-induced muscle loading conditions. In brain-dead patients, Levine et al. reported severe diaphragm muscle fiber atrophy after 18–69 h of mechanical ventilation, whereas pectoralis muscle fibers were entirely preserved [9]. An average 30% loss of the force-generating capacity of the diaphragm has been observed following thoracic surgery with short mechanical ventilation periods (less than 2 h), whereas the contractile performance of latissimus dorsi muscle was preserved [10].
18.3 Exercise-Induced Muscular and Cardiopulmonary Function
Compared with pharmacological interventions, exercise training is currently recognized as one of the most efficient interventions to improve physical and psychological health in patients with cardiovascular, pulmonary, and rheumatic diseases as well as with cancer, obesity, or mental disorders.
18.3.1 Type of Exercise
Physical training programs encompass resistance or strength-type exercises and endurance or aerobic-type exercises. Increasing muscular mass is usually achieved by “resistive work” or static (isometric) contraction without any change in muscle length. In contrast, dynamic (isotonic) muscle actions entail concentric and eccentric contractions leading to muscle shortening and lengthening, respectively.
18.3.2 Mechanism of Exercise-Induced Improvement in Physical Fitness (Fig. 18.3)

Fig. 18.3
Mechanisms of exercise-induced changes in muscle fiber phenotype
In “frail” sarcopenic subjects, resistance exercises (isometric contractions) are particularly effective in (re)building up muscle mass (hypertrophic changes dominates hyperplasia) with significant improvement in strength and joint mobility. In contrast, aerobic exercises lead to a minor increase in muscle mass/strength, but their beneficial health benefits are attributed to attenuation of systemic inflammation, enhanced angiogenesis, and phenotypical changes of cardiac and skeletal myocytes from type IIb into type IIa fibers with increased oxidative capacity (Fig. 18.3). The mechanisms underlying the aerobic exercise-induced increase in VO2max are multifactorial involving partial reversal of endothelial dysfunction and adrenergic receptor responsiveness, higher capillary density, restoration of insulin sensitivity, and enhanced mitochondrial performances owing to tighter coupling between beta-oxidation and the tricarboxylic acid cycle. The enhanced cardiac output and facilitated tissue oxygen diffusion coupled with greater extraction of oxygen by the working muscle all contribute to increase in aerobic capacity after short training periods (Table 18.2).
Table 18.2
Mechanisms of exercise-induced improvement in oxygen transport components
Oxygen transport component | Long term (≥4 weeks) | Short term (<4 weeks) | Comment | |
---|---|---|---|---|
Pulmonary | Respiratory muscles (breathing exercise) | = | = | Respiratory muscle training is associated with reduced dyspnea |
Diffusion capacity | = | = | ||
Airway obstruction, airflow trapping | = | = | Improved by bronchodilators | |
Pulmonary vascular (remodeling) | = | = | ||
Cardiac | Systolic ventricular function (contractility) | ↗or = | ? | |
Diastolic ventricular function (relaxation) | ↗or = | ? | ||
Peak stroke volume | ↗↗ | (↗) | ||
Peak heart rate | ↗↗ | ↗ | ||
Ventilatory equivalents at ventilatory threshold | ↙ | ? | ||
Cardiac Output | ↗↗↗ (♂ > ♀) | ↗ (♂ > ♀) | ||
Tolerance to myocardial ischemia | ↗ | ↗ | ||
Vascular | Endothelial function (NO release) | ↗ | ↗ | |
Arterial stiffness | ↙ | ? | ||
Anti-inflammatory expression | ↗ | ? | ||
Blood | Hemoglobin concentration | ↗ | = | |
All | Ventilatory (anaerobic) threshold | ↗ | ? | |
Skeletal muscle | Arteriovenous oxygen difference | = elderly ♂ ↗elderly ♀ | ||
Capillary density | ↗ | ? | ||
Enzymes for oxidative phosphorylation | ↗↗ | ↗ | ||
Mitochondrial density | ↗↗ | ↗ | ||
Myoglobin concentration | ↗ | ? | ||
Fiber transition to fatigue resistant phenotype (type I to type IIA) | Yes | ? | ||
Muscle mass | ↗ | (↗) |
18.3.2.1 Experimental Data
In several animal models, repeated bouts of intense muscular activity (equivalent to high-intensity interval training [HIIT]) to achieve 80–90 % HRmax or VO2max have demonstrated cardioprotective effects quite similar to ischemic preconditioning. Protective cellular processes in the heart are mediated by sarcolemmal and mitochondrial ATP-sensitive potassium channels, generation of antioxidant molecules (superoxide dismutase, catalase), overexpression of heat shock protein (HSP70, HSP27), and upregulation of autophagic responses (Fig. 18.4). Exercise-induced cardiac mitochondrial adaptations have been shown to result in decreased reactive oxygen species production, increasing the heart’s ability to tolerate high calcium levels and to sustain subsequent acute ischemic events.


Fig. 18.4
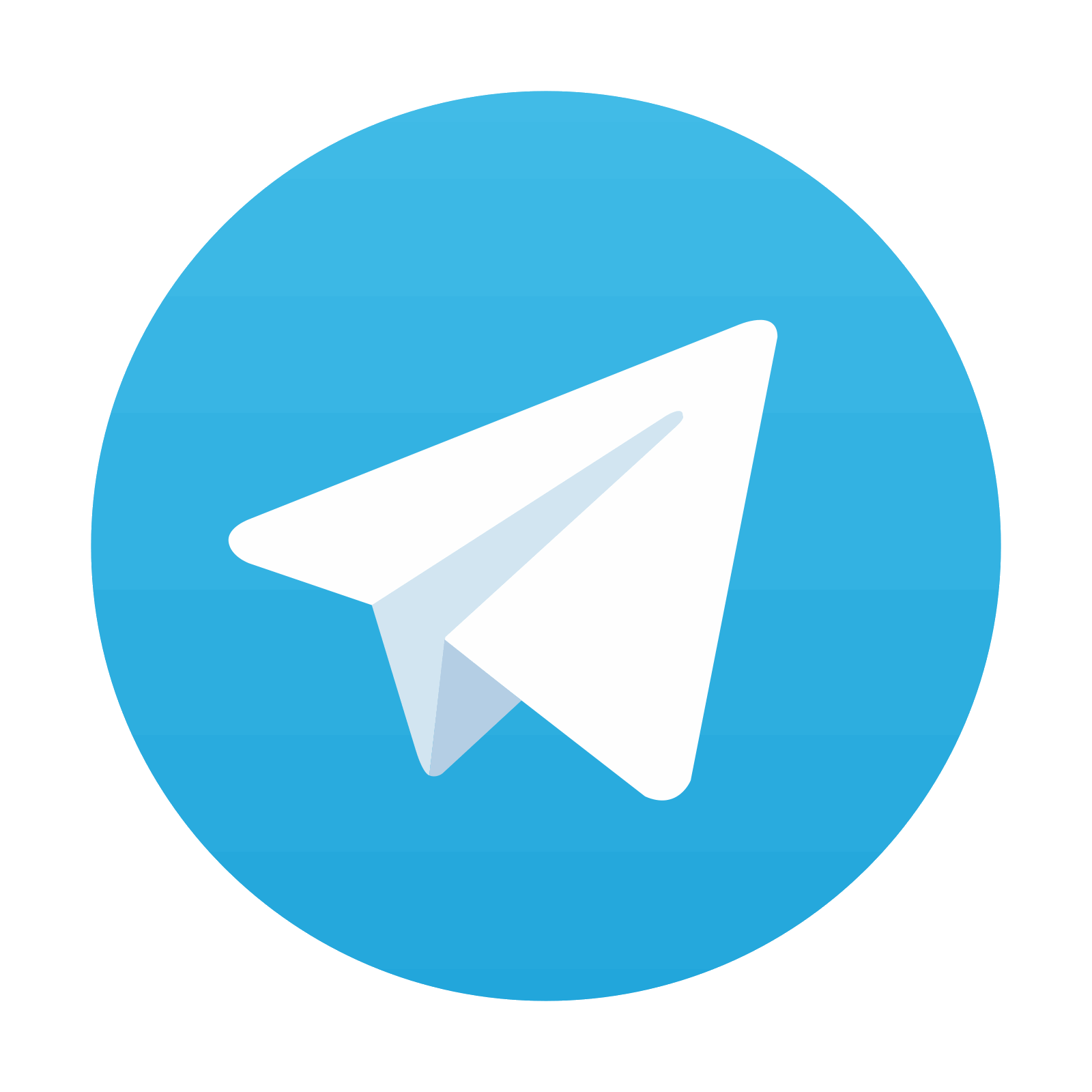
Organ-protective mechanisms induced by aerobic high-intensity training. EPC endothelial progenitor cells; NO nitric oxide; SCDF stromal cell-derived factor; COX-2 cyclooxygenase type 2; PKC protein kinase C; AMPK adenosine monophosphate kinase; ROS reactive oxygen species; AMP, ADP, and ATP adenosine mono-, di-, and triphosphate; mPTP mitochondrial permeability transition pore; mito and sarco KATP mitochondrial and sarcolemmal potassium ATP channel
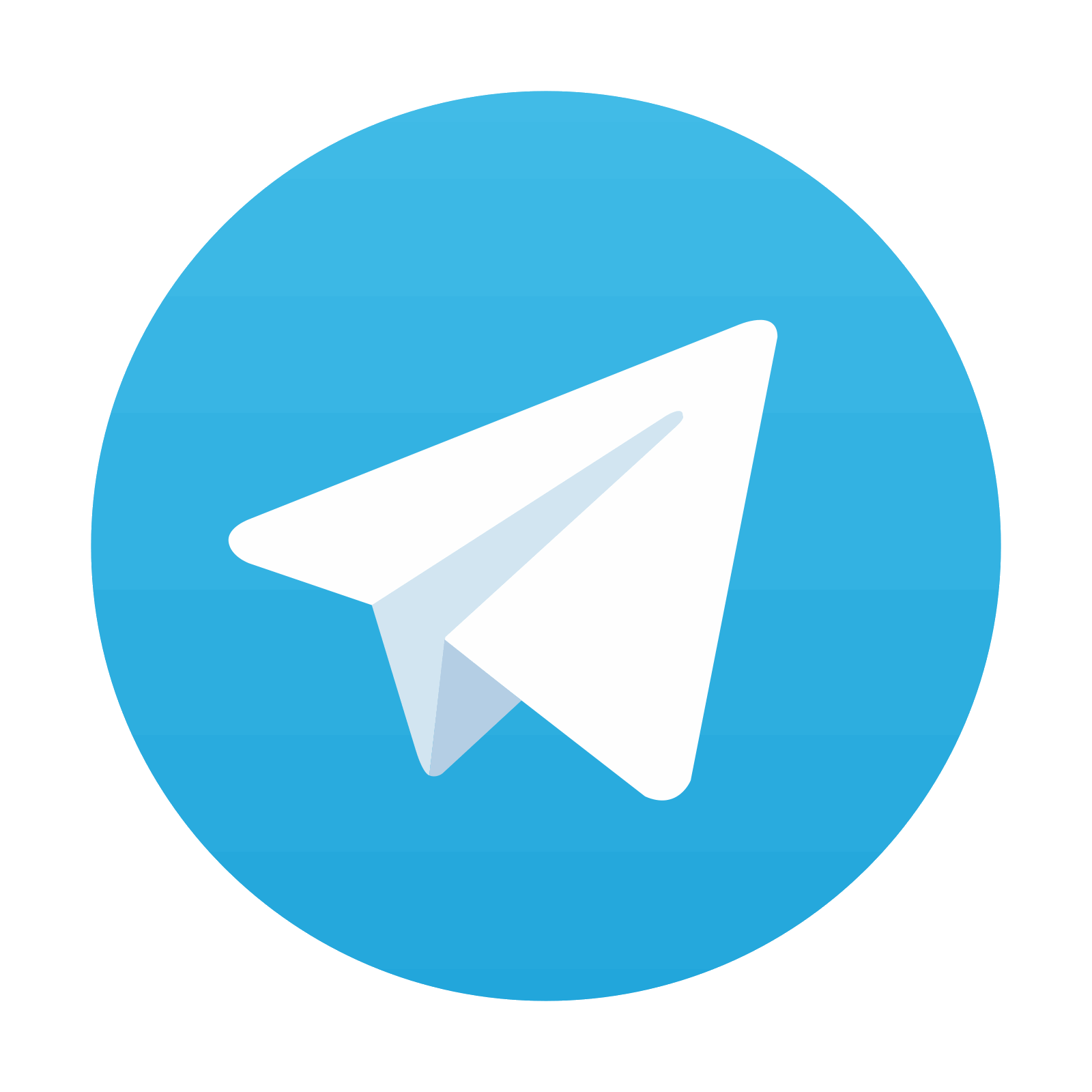
Stay updated, free articles. Join our Telegram channel
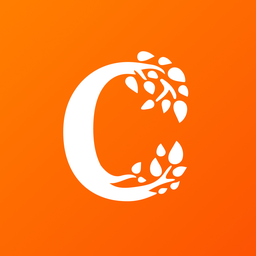
Full access? Get Clinical Tree
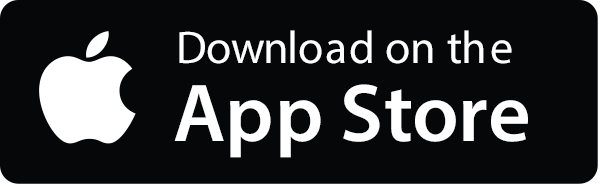
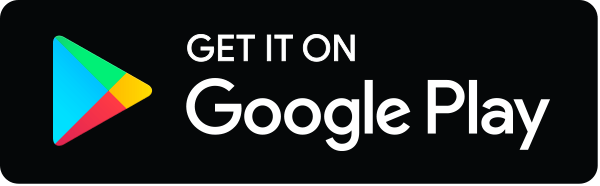
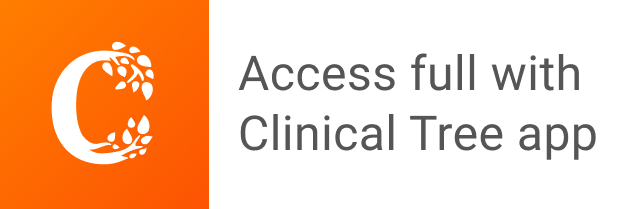