This chapter discusses the fundamental observations that have profoundly changed our view of the adult human heart, resulting in the experimental use of stem/progenitor cells as a potential form of therapy for the failing heart. For nearly a century, the general view was that the heart is a terminally differentiated postmitotic organ in which the number of cardiomyocytes is established at birth, and these cells persist throughout the lifespan of the organ and organism. From birth to adulthood and senescence, the increase in myocardial mass was assumed to be dictated by a parallel increase in volume of cardiomyocytes; the changes in cell size were considered equivalent to the changes in ventricular weight. Myocyte enlargement was regarded as the exclusive mechanism available to the heart to increase its muscle compartment. In the absence of coronary artery disease and other pathologic conditions, the number of cardiomyocytes was thought to remain constant throughout life. Cardiac hypertrophy and its regression were conditioned, respectively, by cellular enlargement and atrophy; the 250- to 300-g human heart was presumed to contain the same number of myocytes present in the markedly hypertrophied heart, weighing 1000 g or more. Myocytes may quadruple their volume, and they modulate their age and mechanical behavior by turnover of their cytoplasmic proteins and mitochondrial organelles. Myocytes may live and function up to 90 years or longer, coinciding with an individual’s lifespan. This notion of cardiac biology has dictated basic and clinical research in the last century.
A series of studies in humans appeared in the late 1990s and early 2000s documenting that cardiac hypertrophy is characterized by a chronic loss of myocytes mediated by cell apoptosis and necrosis, together with an increase in volume and number of parenchymal cells. Myocyte formation, hypertrophy, and death occur in humans with systemic hypertension or aortic stenosis and after acute and chronic myocardial infarction (MI) or idiopathic dilated cardiomyopathy. Similar observations have been made experimentally, strengthening the notion that myocyte growth in its two aspects, hypertrophy and proliferation, and myocyte death in its two forms, apoptosis and necrosis ( Figure 16-1, A ), are important determinants of cardiac mass and function. The plasticity of the myocardium cannot be restricted to cellular hypertrophy but must include myocyte death and regeneration. These three interrelated variables determine the balance between pathologic overloads and the adaptive capacity of the human heart, structurally and functionally. Collectively, these results have offered a more biologically valid interpretation of the growth reserve of the heart and its myocyte population. Activation of the components of the cell cycle machinery, karyokinesis and cytokinesis, have been detected in cardiomyocytes, imposing a reinterpretation of the growth mechanisms of the adult heart. At all ages, the heart contains a pool of replicating myocytes that express the cell cycle proteins CDC6, Ki67, MCM5, phospho-H3, or aurora B kinase ( Figure 16-1, B ). But whether dividing myocytes are transit-amplifying cells generated by commitment of endogenous or exogenous stem cells, or whether they constitute a pool of cells that retains the ability to reenter the cell cycle and divide, remains unclear. The possibility has also been raised that myocytes possess a certain degree of developmental plasticity and are able to dedifferentiate, acquire a proliferative state, multiply, and form functionally competent cells. These findings challenge stem cell differentiation as the predominant source of myocytes, pointing to alternative mechanisms of myocyte renewal in the adult heart. However, these studies fell short in providing evidence in favor of the formation of cardiomyocytes independently from stem cell commitment. Therefore, stem cell–based therapy is, at present, the most promising option for the potential reconstitution of damaged myocardium.

Circulating Progenitor Cells and Myocardial Regeneration
The documentation that stem/progenitor cells are present within the myocardium raised the question as to whether these primitive cells reside in the heart or derive from distant organs such as the bone marrow. The first evidence supporting the notion that the heart is a stem cell–regulated organ was provided by the identification of male cells in female hearts transplanted in male recipients. In these cases of sex-mismatched cardiac transplants, the female heart in a male host had a significant number of Y chromosome–positive myocytes and coronary vessels. Although discrepancies exist among groups in terms of the magnitude of chimerism, these results documented that male cells colonize the female heart and differentiate into cardiovascular structures ( Figure 16-1, C ). A comparison has also been made between the degree of chimerism of cardiomyocytes and coronary vessels in cardiac allografts and in hearts of patients who received allogeneic bone marrow transplants. In the latter case, only 2% to 5% chimeric myocytes were detected, but 14% to 16% chimeric myocytes and endothelial cells were observed in the transplanted heart. Host cells may migrate from the residual atrial stumps to the donor heart or may reach the myocardium through the circulation.
In the presence of tissue injury, the bone marrow may sense distant signals that favor the translocation of bone marrow cells (BMCs) to the site of damage, promoting organ repair. The physiologic relevance of circulating stem/progenitor cells to hematopoiesis is well known. Migration represents a fundamental step in the determination of hematopoietic stem cell (HSC) fate through the relocation of daughter HSCs to distinct marrow niches. A similar phenomenon may be involved in the contribution of circulating HSCs and BMCs to the regeneration of injured nonhematopoietic organs ( Figure 16-2 ).

Migration, homing, and proliferation of endothelial progenitor cells (EPCs) in ischemic regions of the heart and the hind limb lead to de novo formation of vascular structures. In addition, circulating EPCs participate, together with resident vascular progenitors, in the reendothelization of the damaged vessel wall. EPCs are a subset of bone marrow mononuclear cells, which give rise to functionally competent endothelial cells and result in neovascularization of ischemic parenchyma. The bone marrow mononuclear cells, which express the CD34 antigen, share some of the properties of EPCs. However, CD34-positive cells exhibit superior efficacy in the preservation of myocardial integrity and function after infarction than unselected circulating mononuclear cells.
An increased number of circulating EPCs and CD34-positive cells have been reported during the early phases of MI, although these cells have partly lost their functional integrity. Patients with type 1 and type 2 diabetes have a reduced pool of EPCs, a decrease proportional to the severity of the diabetic vasculopathy. The functional impairment of EPCs is conditioned by dysregulation of nitric oxide synthase, enhanced production of reactive oxygen species, and activation of proinflammatory protein kinases. Other cardiovascular risk factors—including hypercholesterolemia, hypertension, and smoking—are coupled with dysfunctional EPCs. In addition, chronologic age in healthy individuals and in patients with coronary artery disease is associated with decreased number and function of circulating EPCs. Aging is accompanied by shortening of telomeres in leukocytes and other bone marrow–derived cells, and coronary artery disease and heart failure further enhance telomere attrition. Telomeric shortening in patient-derived EPCs conditions their reduced migratory response to vascular endothelial growth factor (VEGF) and stromal-derived factor-1 (SDF-1), suggesting that age-associated telomere erosion contributes to impaired EPC function. Collectively, these observations indicate that cardiovascular risk factors profoundly affect the therapeutic benefit of EPCs.
Hematopoietic Stem Cell Transdifferentiation
In the past decade, major discoveries have been made concerning the biology of adult HSCs. They can differentiate into cell lineages distinct from the organ in which they reside and into cells derived from a different germ layer. These properties were considered to be restricted to embryonic stem cells, and because of their dramatic biologic and clinical implications, they triggered a vigorous debate in the scientific community. The controversy reached unexpected levels of intensity when HSCs were shown to be able to migrate to sites of injury, repairing damage in various organs and in the heart in particular. The heart has always been viewed as a nonpermissive organ for exogenous and endogenous tissue regeneration. Studies presenting positive results were confronted by negative reports, which challenged stem cell plasticity and the emerging new paradigm of organ and organism homeostasis and repair. However, molecular and genetic tools have been used to obtain unequivocal evidence of HSC transdifferentiation; HSCs acquire the cardiomyocyte and vascular lineages, promoting the structural and functional recovery of the damaged heart.
HSCs express the receptor tyrosine kinase c-kit, and when injected in the border zone of a MI or mobilized systemically into the circulation with cytokines, HSCs lead experimentally to the repair of the necrotic tissue and the formation of functionally competent myocardium. These observations were confirmed in multiple laboratories and argue in favor of the differentiation of bone marrow–derived cells into the myogenic and vascular cell phenotypes as the mechanism of cardiac repair ( Figure 16-3, A ). The formed cardiac cells in female infarcted hearts carry the fluorescent tag and the Y chromosome of the injected enhanced green fluorescent protein (EGFP)-positive male HSCs ( Figure 16-3, B ).

In addition, BMC transdifferentiation was shown by the delivery to the infarcted heart of c-kit–positive HSCs carrying a reporter gene under the control of a myocyte-specific promoter ( Figure 16-3, C ). These findings, and the paucity of effective new drugs for the treatment of heart failure, have prompted cardiologists to the rapid implementation of bone marrow–derived cells in the management of the infarcted human heart. However, a mixed population of BMCs, rather than a highly purified stem cell pool, has commonly been used for the restoration of the injured myocardium. Thus far, c-kit–positive HSCs have not been used in clinical trials.
If c-kit–positive HSCs attain the properties of cardiac stem cells (CSCs) when they home to the myocardium and give rise to cardiomyocytes and coronary vessels, the concept of transdifferentiation has to be accepted. And understanding whether CSCs and HSCs are separate stem cell categories becomes extremely important. Hypothetically, stem cells that reside in the heart should be more effective in making new myocardium than stem/progenitor cells from other organs, including the bone marrow. CSCs are programmed to generate heart structures and, upon activation, can rapidly form parenchymal cells and coronary vessels, possibly rescuing the failing heart. Conversely, HSCs have to acquire a different phenotype, which necessitates chromatin remodeling and reprogramming of genes before they transdifferentiate and create functionally competent myocardium. To date, the HSC appears to be the most versatile stem cell in crossing lineage boundaries and the most prone to break the law of tissue fidelity.
In addition to HSCs, the bone marrow contains a population of mesenchymal stromal cells (MSCs). The high degree of plasticity of MSCs supports their phenotypic flexibility and the ability to switch from one cell type to another. Because MSCs are multipotent and can be easily expanded in culture, there has been significant interest in their potential for tissue regeneration. MSCs appear to possess a capacity for cardiac repair that exceeds their ability to differentiate into cardiomyocytes. Recently, it has been shown that bone marrow–derived MSCs injected in a large animal model of MI promote cardiac repair by activating resident c-kit–positive CSCs and by enhancing the proliferation of host transit amplifying myocytes. The interaction between recipient CSCs and delivered MSCs may reflect the cell-to-cell communication, which is operative within stem cell niches.
Bone Marrow Cells and Clinical Studies
Shortly after the experimental evidence that HSCs induce myocardial regeneration after infarction, pilot clinical studies documented that intracoronary infusion of BMCs is feasible and may improve functional recovery in patients with acute myocardial infarction (AMI). The Transplant of Progenitor Cells and Regeneration Enhancement in Acute Myocardial Infarction (TOPCARE-AMI) trial was the first randomized study to investigate the outcome of this approach in 59 patients with successfully reperfused AMI. At 5 years, the results of the trial demonstrated the long-term safety of intracoronary BMC administration and suggested that this form of cell therapy has a beneficial effect on left ventricular (LV) function. Magnetic resonance imaging (MRI) analysis of 31 patients confirmed a significant 11% increase in LV ejection fraction (LVEF) and a reduction in functional infarct size. LV end-systolic volume (LVESV) remained stable, but LV end-diastolic volume (LVEDV) enlarged.
The first double-blind, placebo-controlled, randomized multicenter Reinfusion of Enriched Progenitor Cells and Infarct Remodeling in Acute Myocardial Infarction (REPAIR-AMI) study was then designed. Following successful reperfusion of AMI, patients underwent bone marrow aspiration, and a heterogeneous population of mononuclear cells containing a small fraction of stem/progenitor cells was obtained by Ficoll gradient. These cells were infused intracoronarily within the segment containing the stent. With respect to controls, LVEF increased by 2.8% points at 12 months. In patients with baseline LVEF less than the median, cell therapy was associated with a 6.6% points improvement in LVEF, which was coupled with abrogation of LVESV expansion and attenuation in LVEDV enlargement. The incidence of the prespecified combined clinical endpoints of death, reinfarction, and coronary revascularization was significantly lower in the BMC-treated patients than in placebo controls, and these positive effects persisted at 2 years.
Concurrently, a similar study, the Autologous Stem Cell Transplantation in Acute Myocardial Infarction (ASTAMI) trial, was conducted in patients with anterior-wall AMI. At 6-month and 3-year follow-up, the 7.6% points increase in LVEF was similar in control and stem cell–treated groups. End-diastolic volume, infarct size, and global LV systolic function measured by echocardiography and MRI did not differ in control and stem cell–treated patients. A small improvement in exercise time was observed in treated patients 3 years after cell infusion. The difference between the REPAIR-AMI and ASTAMI trial has been attributed to the protocols used for the isolation and storage of BMCs. Compared with BMCs used in the REPAIR-AMI, those used in the ASTAMI trial had a decreased migratory capacity in vitro and a reduced vasculogenic potential in vivo.
Isolation procedures, sorting protocols, and preservation media have a major impact on the bioactivity of progenitor cells. The importance of these variables became apparent when the functional properties of BMCs were found to parallel the degree of contractile improvement of patients enrolled in the REPAIR-AMI trial. The level of contamination of the final BMC product with red blood cells (RBCs) correlated with the reduced recovery of LVEF. The presence of RBCs was associated with lower BMC viability, decreased colony formation and invasion ability in vitro, and impaired capacity to form new capillaries in vivo. These factors conditioned the therapeutic efficacy of BMCs, diminishing the extent of LVEF improvement.
The conflicting results of the REPAIR-AMI and ASTAMI trials are just one example of the controversy in the field. The substantial disparity among studies was investigated by designing meta-analyses that involved subgroups of trials, in which one or more unifying parameters were identified: route of delivery, type of cardiac disease, age and gender of the patients, and presence of comorbidities. Intracoronary injection of BMCs in patients with AMI was found to be safe and effective, with an average 3.79% points increase in ejection fraction with respect to baseline. However, the persistence of the beneficial effects of intracoronary injection of BMCs over time remained controversial. The Bone Marrow Transfer to Enhance ST-Elevation Infarct Regeneration (BOOST) trial showed that BMC therapy did not improve LVEF at 5-year follow-up, whereas the Treatment of Hyponatremia Based on Lixivaptan in New York Hospital Association (NYHA) Class III/IV Cardiac Patient Evaluation (BALANCE) study demonstrated a long-term benefit of autologous cell administration. Patients enrolled in the BOOST trial had ST-segment elevation myocardial infarction (STEMI) and relatively preserved systolic function. A single intracoronary infusion of BMCs led to a significant (6% points) improvement in LVEF at 6 months, which declined to 2.8% points at 18 months. After 5 years, major adverse cardiac events occurred with similar frequency in cell-treated and untreated patients. With respect to baseline, LVEF by MRI decreased 3.3% points in controls and by 2.5% points in BMC-treated patients. When the subgroup of patients with infarct transmurality higher than the median was evaluated independently, the initial benefit of BMC administration persisted throughout the 61-month study period.
In the BALANCE trial, cells infused directly into the infarct-related artery led to a significant improvement of contractility in the border zone of the infarct and to reduction of arrhythmogenic events at 12 and 60 months. Compared with controls, mortality rate was significantly reduced in BMC-treated patients. Because of the conflicting results of the BOOST and BALANCE trials, meta-analyses have been conducted to address specifically the question concerning the durability of BMC therapy. The evaluation of randomized controlled trials with 1 year or more of follow-up documented a significant 4.37% points increase of LVEF in BMC-treated patients with respect to controls. This functional improvement was accompanied by reductions in LVEDV, LVEDS, and infarct size. By meta-regression analyses, BMC therapy was found to be more successful in older and diabetic patients and less effective in males.
BMCs have been administered more frequently via the intracoronary route; however, intramyocardial BMC transplantation was introduced during cardiac artery bypass graft (CABG) surgery in about 20 trials. In six cases, the homogeneity among studies in patients affected by chronic ischemic cardiomyopathy was considered sufficient for a meaningful comparison. With respect to controls, in which CABG was not combined with cell infusion, BMC delivery resulted in a 5.4% points increase in LVEF and a small decrease in LVEDV. Despite the relative homogeneity of these six clinical trials, a relevant source of inconsistency has been found in the type and dose of delivered cells. In three clinical trials, CD133-positive or CD34-positive BMCs were used; these cell pools are highly enriched for stem/progenitor cells of hematopoietic and endothelial lineages.
Patients affected by dilated cardiomyopathy have been enrolled in a few pilot studies. The prospective, open-label Transplantation of Progenitor Cells and Recovery of Left Ventricular Function in Patients with Nonischemic Dilatative Cardiomyopathy (TOPCARE-DCM) trial involved 33 patients with LVEF lower than 40% and left ventricular end-diastolic dimension (LVEDD) greater than 60 mm. With respect to baseline, a measurable improvement of regional wall motion was detected at 3 months and persisted at 12 months, when an absolute 3.2% points increase in LVEF was accompanied by a reduction in serum levels of N-terminal prohormone brain natriuretic peptide (NT-proBNP). No significant changes were seen in LVEDV and LVESV.
Similar results were obtained in a recent open-label randomized study that involved 55 patients with dilated cardiomyopathy and severe ventricular dysfunction (LVEF <30%). Patients received CD34-positive cells by intracoronary infusion. This intervention resulted at 3 months in a 5% points increase in LVEF above control value, which persisted at 12 months. Cell therapy did not decrease LVEDV; however, untreated patients showed expansion in cavitary volume over time. Cell administration improved exercise capacity and significantly reduced the serum levels of NT-proBNP. The combined cardiac mortality/heart transplantation rate was significantly lower in the BMC-treated group, and the excellent safety profile of these two pilot trials supports the rationale for larger, multicenter studies.
Patients with refractory angina have been enrolled in a double-blind, randomized, placebo-controlled dose-escalating study. After 5 days of treatment with granulocyte colony-stimulating factor (G-CSF), CD34-positive cells were immunosorted and injected intramyocardially. Efficacy parameters that included angina frequency, nitroglycerine usage, exercise time, and Canadian Cardiovascular Society class showed trends that favored CD34-positive cell-treated patients versus controls. A larger phase IIb study is currently ongoing.
Allogeneic and autologous MSCs have been used in small clinical trials, with encouraging results. A safety study based on the intravenous delivery of allogeneic MSCs was conducted in 53 reperfused AMI patients. Global symptom score in all patients and ejection fraction in the subset of subjects with anterior AMI were significantly better in MSC-treated versus placebo controls. Based on a large amount of preclinical data obtained in porcine models of ischemic cardiomyopathy, a phase I pilot study was conducted in eight patients who received intramyocardial injection of autologous mononuclear or mesenchymal BMCs in the LV scar and border zone. This safe therapeutic strategy produced functional recovery of the scarred myocardium and reverse remodeling of the LV. At 1 year, MRI analysis demonstrated a significant decrease in LVEDV and infarct size, and a trend toward decreased LVESV was observed. Improved regional function of the infarct zone strongly correlated with reduction of LVEDV and LVESV.
The mechanisms involved in the positive impact of BMC therapy on patients remains to be identified. The impossibility to permanently label the cells to be delivered and the difficulty to obtain cardiac biopsies to assess parameters consistent with myocardial regeneration leaves uncertain our understanding of the cellular processes mediating partial myocardial recovery. Measurements of coronary flow suggest that vasculogenesis may be operative, but the contribution of de novo myocyte formation is uncertain. Reductions in infarct scar size speak in favor of myocardial regeneration, although unequivocal data have not been obtained as yet. The recent identification of CSCs has shifted the attention to endogenous cell mechanisms as a novel target of cell therapy for the failing heart.
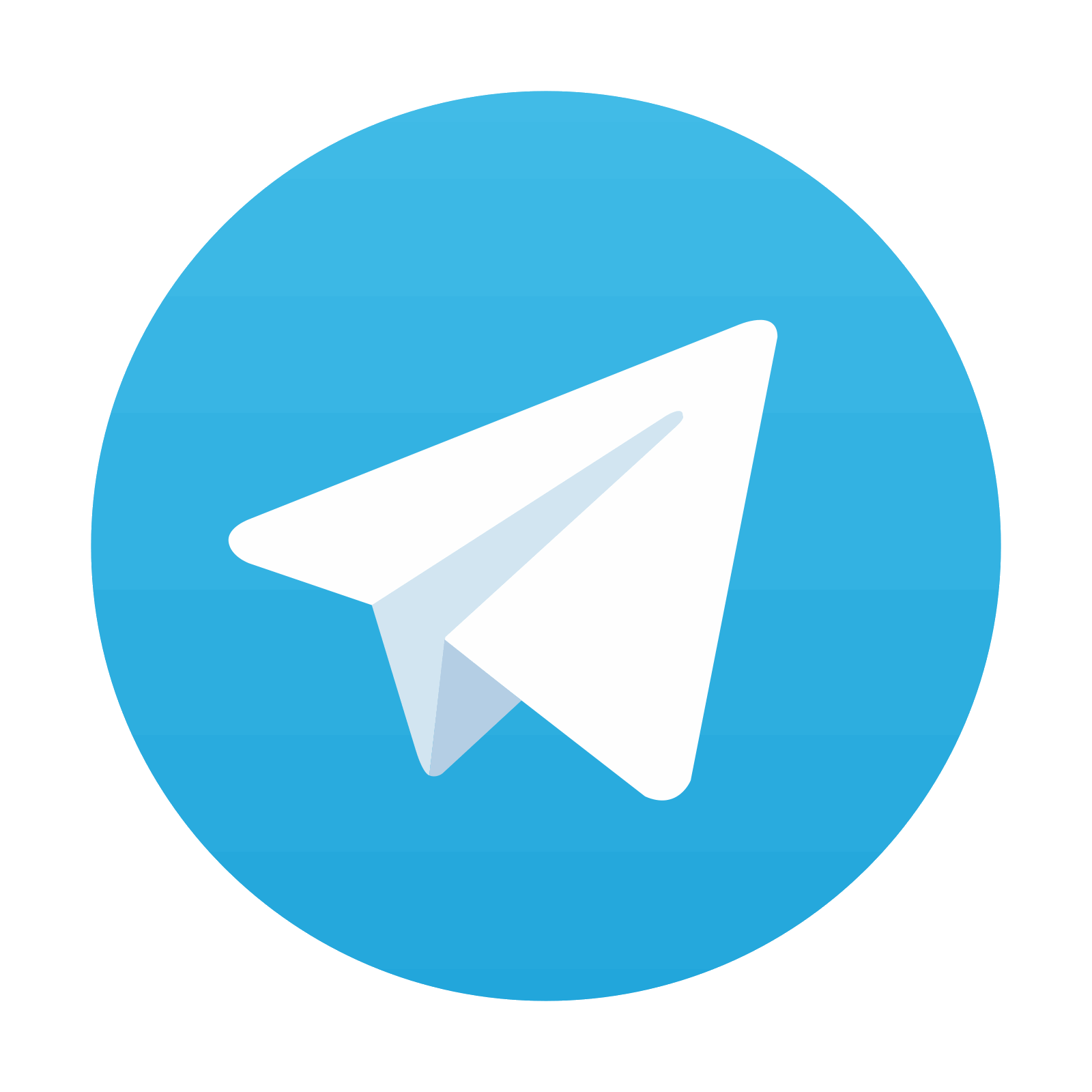
Stay updated, free articles. Join our Telegram channel
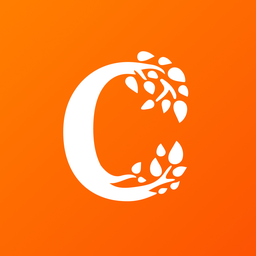
Full access? Get Clinical Tree
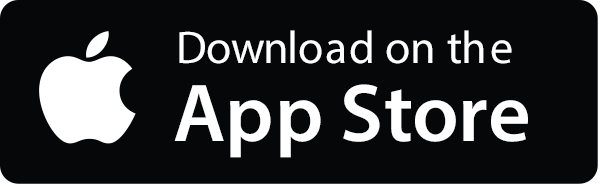
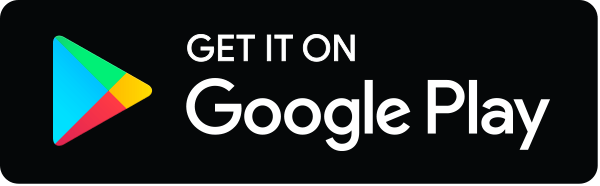