Accreditation Statement
The American Society of Echocardiography is accredited by the Accreditation Council for Continuing Medical Education to provide continuing medical education for physicians.
The American Society of Echocardiography designates this educational activity for a maximum of 1.5 AMA PRA Category 1 Credits ™. Physicians should only claim credit commensurate with the extent of their participation in the activity.
ARDMS and CCI recognize the ASE’s certificates and have agreed to honor the credit hours toward their registry requirements for sonographers.
The American Society of Echocardiography is committed to ensuring that its educational mission and all sponsored educational programs are not influenced by the special interests of any corporation or individual, and its mandate is to retain only those authors whose financial interests can be effectively resolved to maintain the goals and educational integrity of the activity. Although a monetary or professional affiliation with a corporation does not necessarily influence an author’s presentation, the Essential Areas and policies of the ACCME require that any relationships that could possibly conflict with the educational value of the activity be resolved prior to publication and disclosed to the audience. Disclosures of faculty and commercial support relationships, if any, have been indicated.
Target Audience
This activity is designed for all cardiovascular physicians and cardiac sonographers with a primary interest and knowledge base in the field of echocardiography; in addition, residents, researchers, clinicians, intensivists, and other medical professionals with a specific interest in cardiac ultrasound will find this activity beneficial.
Target Audience
This activity is designed for all cardiovascular physicians and cardiac sonographers with a primary interest and knowledge base in the field of echocardiography; in addition, residents, researchers, clinicians, intensivists, and other medical professionals with a specific interest in cardiac ultrasound will find this activity beneficial.
Objectives
Upon completing the reading of this article, participants will better be able to:
- 1.
Review the techniques for optimizing imaging and quantifying cardiac structures in the pediatric population.
- 2.
Explain the importance of adjusting measurements of cardiovascular structures for the effects of body size.
- 3.
Identify the pediatric quantification protocols for the pulmonary veins, systemic veins, atria and atrioventricular valves.
- 4.
Recognize and apply the recommended echocardiographic methods for the evaluation of left and right ventricular size and systolic function.
- 5.
Define the optimal views and the appropriate anatomic sites for correct measurement of the proximal ascending, proximal and distal arch, and descending aorta.
- 6.
Employ appropriate transducer position and Doppler technique for the anatomic and hemodynamic interrogation of the aorta and aortic valve and the pulmonary artery and the pulmonary valve.
Author Disclosure
The authors of this article reported no actual or potential conflicts of interest in relation to this activity.
The ASE staff and ASE ACCME/CME reviewers who were involved in the planning and development of this activity reported no actual or potential conflicts of interest: Chelsea Flowers; Rebecca T. Hahn, MD, FASE; Cathy Kerr; Priscilla P. Peters, BA, RDCS, FASE; Rhonda Price; and Cheryl Williams.
The following members of the ASE Guidelines and Standards Committee, JASE Editorial staff and ASE Board of Directors reported no actual or potential conflicts of interest in relation to this activity: Deborah A. Agler, RCT, RDCS, FASE; J. Todd Belcik, BS, RDCS, FASE; Renee L. Bess, BS, RDCS, RVT, FASE; Farooq A. Chaudhry, MD, FASE; Robert T. Eberhardt, MD; Benjamin W. Eidem, MD, FASE; Gregory J. Ensing, MD, FASE; Tal Geva, MD, FASE; Kathryn E. Glas, MD, FASE; Sandra Hagen-Ansert, RDCS, RDMS, MS, FASE; Rebecca T. Hahn, MD, FASE; Jeannie Heirs, RDCS; Shunichi Homma, MD; Sanjiv Kaul, MD, FASE; Smadar Kort, MD, FASE; Peg Knoll, RDCS, FASE; Wyman Lai, MD, MPH, FASE; Roberto M. Lang, MD, FASE; Steven Lavine, MD; Steven J. Lester, MD, FASE; Renee Margossian, MD; Victor Mor-Avi, PhD, FASE; Sherif Nagueh, MD, FASE; Alan S. Pearlman, MD, FASE; Patricia A. Pellikka, MD, FASE; Miguel Quinones, MD, FASE; Brad Roberts, RCS, RDCS; Beverly Smulevitz, BS, RDCS, RVS; Kirk T. Spencer, MD, FASE; J. Geoffrey Stevenson, MD, FASE; Wadea Tarhuni, MD, FASE; James D. Thomas, MD; Neil J. Weissman, MD, FASE; Timothy Woods, MD; and William A. Zoghbi, MD, FASE.
The following members of the ASE Guidelines and Standards Committee, JASE Editorial staff and ASE Board of Directors reported a relationship with one or more commercial interests. According to ACCME policy, the ASE implemented mechanisms to resolve all conflicts of interest prior to the planning and implementation of this activity. Theodore Abraham, MD, FASE receives honoraria and research grant support from GE Healthcare. Patrick D. Coon, RDCS, FASE is on the speaker’s bureau for Philips Medical. Victor G. Davila-Roman, MD, FASE is a consultant for St. Jude Medical, AGA Medical, Medtronic, CoreValve, Boston Scientific Corporation, and Sadra Medical. Elyse Foster, MD receives grant support from Abbott Vascular Structural Heart, EBR Systems, Inc., and Boston Scientific Corporation. Julius M. Gardin, MD, FASE is a consultant/advisor to Arena Pharmaceuticals. Jeffrey C. Hill, BS, RDCS, FASE receives grant/research support from Toshiba America Medical Systems and Philips; is a consultant to Medtronic; and is on the speaker’s bureau for Philips. Martin G. Keane, MD, FASE is a consultant/advisor to Pfizer, Inc. and Otsuka Pharmaceuticals. Gilead I. Lancaster, MD, FASE owns stock in, and is a consultant/advisor to, Cardiogal. Jonathan R. Linder, MD, FASE is a consultant/advisor to VisualSonics. Carol C. Mitchell, PhD, RDMS, RDCS, RVT, RT(R), FASE is a speaker and consultant for GE Healthcare. Marti McCulloch, MBA, BS, RDCS, FASE is a speaker for Lantheus and advisor/consultant for Siemens. Tasneem Z. Naqvi, MD, FASE is a consultant/advisor to Edwards Lifesciences and St. Jude Medical, and receives grant support from Medtronic and Actor Medical. Kofo O. Ogunyankin, MD, FASE is on the speaker’s bureau for Lantheus. Vera Rigolin, MD, FASE is on the speaker’s bureau for Edwards Lifesciences and St. Jude Medical. Lawrence G. Rudski, MD receives grant support from Genzyme and owns stock in Abbott Labs, Hospira, Johnson and Johnson, and Medtronic. Stephen G. Sawada, MD owns stock in GE Healthcare. Alan D. Waggoner, MHS, RDCS is a consultant/advisor for Boston Scientific Corporation and St. Jude Medical, Inc.
Estimated Time to Complete This Activity: 1.5 hours
Table of Contents
Introduction 466
Optimization Techniques in Imaging and Doppler Evaluation 466
Principles and Methods for Adjusting Measurements of Cardiovascular Structures for the Effects of Body Size 467
Pediatric Quantification Protocols 468
Pulmonary Veins, Systemic Veins, and Atria 468
Atrioventricular Valves 470
Left Ventricle 475
Right Ventricle 481
Ventricular Outflow Tracts and Semilunar Valves 484
Aorta, Coronary Arteries, and Pulmonary Arteries 487
Conclusions and Limitations 490
References 490
Introduction
Echocardiographic quantification is crucial in the diagnosis and management of patients with acquired and congenital heart disease (CHD). The American Society of Echocardiography (ASE) and the European Association of Echocardiography have published recommendations on how to measure the size and function of cardiovascular structures in adults, providing reference limits to distinguish normal from abnormal values. Identifying an abnormal measurement helps assess the effect of a disease on the size of a cardiovascular structure, determine when intervention may be necessary, and monitor the effect of the intervention. Examples in which these standards are useful include aortic root dilation in Marfan syndrome and left ventricular (LV) dilation with a ventricular septal defect. However, the size of cardiovascular structures is influenced not only by the hemodynamics of disease states and their treatments but also by confounding factors. such as growth, age, genes, gender, race, body composition, basal metabolic rate, hematocrit, exercise, and altitude.
Aside from abnormal hemodynamics, body size is the most powerful determinant of the size of cardiovascular structures: all cardiovascular structures increase in size relative to somatic growth, a phenomenon known as cardiovascular allometry. Expressing measurements relative to body size allows a meaningful distinction between normal and abnormal values in children. It does require the collection of quantitative data from a normal pediatric population to function as the standard against which all measurements are compared. Because there must be agreement on how to measure the size of each cardiovascular structure, this document describes the recommended protocols for the morphometric evaluation of the heart in children with or without CHD, and recommendations at the end of each section refer to measurements that may be useful for the creation of a pediatric normative database. However, the Pediatric Measurements Writing Group emphasizes that the recommended measurements are those that can be performed in a pediatric examination and not necessarily those that must be part of the study.
Optimization Techniques in Imaging and Doppler Evaluation
Standard views are often categorized as “long axis” or “short axis”, and these are described in Table 1 . General optimization techniques in two-dimensional (2D) imaging have been outlined previously for children. Several technical factors can influence the accuracy of spatial measurements: (1) axial resolution parallel to the ultrasound beam is superior to lateral resolution perpendicular to the beam, so views allowing for linear axial measurements are better than those for which only lateral measurements are available (parasternal views are better than apical views for the aortic annulus); (2) lateral resolution degrades with increasing distance secondary to beam spread, so the transducer should be positioned as close as possible to a structure when only a lateral measurement is available; and (3) for large image depths, the ultrasound resolution often exceeds the pixel resolution of the image display, so decreasing the image depth or magnifying the region of interest can often alleviate the limitations of the monitor resolution.
Recommended terminology | Axis designation | Alternate terminology |
---|---|---|
Subxiphoid long-axis | Long axis of left ventricle | Subcostal long-axis Subcostal transverse |
Subxiphoid short-axis | Short axis of left ventricle | Subcostal short-axis Subcostal sagittal |
Apical 4-chamber | Long axis of LV outflow tract | |
Apical 3-chamber | Long axis of LV outflow tract | Apical long-axis |
Apical 2-chamber | Long axis of LV outflow tract | |
Parasternal long-axis | Long axis of LV outflow tract | Left parasternal long-axis |
Parasternal short-axis | Short axis of left ventricle | Left parasternal short-axis |
High left parasternal | Long axis of MPA-LPA continuation | High left parasagittal Ductal |
Suprasternal long-axis | Long axis of ascending and proximal descending Ao displaying aortic arch | Suprasternal aortic arch Suprasternal sagittal |
Suprasternal short-axis | Short axis of ascending Ao | Suprasternal transverse |
Right parasternal | Long axis of SVC and IVC | High right parasternal Right sternal border Right sternal border long-axis Right sternal border sagittal |
Quantitative assessment of each structure should be performed in multiple views, and orthogonal planes should be used for noncircular structures such as the atrioventricular (AV) valves. Early reports based on M-mode echocardiography recommended measurements from the leading edge of the near-field reflector to the leading edge of the far-field reflector, and normative data for the proximal aorta in adults have involved leading edge–to–leading edge measurements. However, current guidelines for chamber, annular, and vessel quantification involve measurements of intraluminal dimensions from one inner edge to the opposite inner edge. In addition, published pediatric normative databases based on 2D echocardiography have used inner edge–to–inner edge measurements of vessel diameters. There are two important caveats with these measurements: vascular diameters should be perpendicular to the long axis of the vessel, and valvar and vascular diameters should be measured at the moment of maximum expansion. In other words, the inferior vena cava (IVC) diameter should be measured during exhalation, the mitral valve (MV) and tricuspid valve (TV) annular diameters in diastole, and the aortic valve (AoV) and pulmonary valve (PV) annular diameters as well as arterial diameters in systole. These recommendations are based on hemodynamic considerations, correspond to the methodologies used in published pediatric normative databases, and often differ from the quantification approach used in adults.
General optimization techniques in Doppler echocardiography have been outlined previously for adults, and their utility must address the abnormal valve and vessel positions and unusual flow jets in patients with CHD. Color mapping should precede spectral Doppler interrogation to identify the direction of flow. The audio feature can help optimize alignment, especially given the unpredictable orientation of flow jets in the third dimension. Doppler waveforms should be displayed at a sweep speed of 100 to 150 mm/s to discriminate temporal changes in the velocity flow profile, particularly in children with high heart rates. Simultaneous electrocardiographic display helps correlate the timing of flow with electrical events. Doppler gain and power settings should be optimized to depict the outer edge of the brightest spectral Doppler envelope; only well-defined envelopes should be measured, and “fuzz” or “feathering” beyond modal velocities should be excluded. Mean gradients calculated from the velocity-time integral (VTI) or area under the velocity curve should be measured from valve opening to closure at the AV and semilunar valves and throughout the cardiac cycle within a blood vessel or at an interatrial communication, incorporating the zero velocity during periods of absent flow. All Doppler measurements should be averaged over 3 consecutive cardiac cycles to account for respiratory variation.
Principles and Methods for Adjusting Measurements of Cardiovascular Structures for the Effects of Body Size
The first step in adjusting for body size involves developing a mathematical description of the mean behavior of the measurement within a normal pediatric population. Ideally, this is based on physiologic principles. Body surface area (BSA) appears to be a better parameter of somatic growth in normal children than height or weight alone. Published equations to calculate BSA often produce variable results, particularly at lower height and weight values, and some are derived from data that do not include children. The Haycock formula (BSA [m 2 ] = 0.024265 × weight [kg] 0.5378 × height [cm ] 0.3964 ) appears to provide the best correlation between BSA and the size of cardiovascular structures (compared with the formulas of DuBois and DuBois, Dreyer and Ray, and Boyd ) and is recommended for calculating BSA. Because of the linear relationship between cardiac output and BSA and the mostly linear relationship between cardiac output and the size of cardiovascular structures, “indexing” the size of structures to BSA has become a fairly common practice. However, assuming that BSA is linearly related to length, area, and volume measurements is mathematically impossible. In addition, BSA-adjusted measurements often manifest a persistent dependence on BSA: the mean value of the BSA-adjusted measurement and the distribution of values around the mean change with increasing BSA (a phenomenon of changing or nonconstant variance known as heteroscedasticity).
Once the mathematical relationship between a measurement and BSA has been determined, the next step involves the confidence intervals and the problem of heteroscedasticity. One approach to find a mathematical descriptor that maintains a stable and constant variance across the range of body sizes might involve transformation of the measurements and/or BSA within a linear or nonlinear regression equation. For example, physiologic principles suggest that distances can be adjusted or normalized to the square root of BSA, areas to BSA, and volumes to BSA 1.5 . This approach results in a mostly linear relationship between the measurement and the transformed BSA, but it does not eliminate the problem of heteroscedasticity; variance continues to be affected by changes in body size. Another example involves performing a logarithmic transformation of the measurements to minimize the problem of heteroscedasticity. However, this method does not effectively describe the population at minimum and maximum BSA values, nor does it fully eliminate heteroscedasticity. There is also no fundamental physiologic explanation for this approach.
An increasingly popular approach in pediatric cardiology to account for the effects of body size and age has been the use of Z scores. Calculation of Z scores involves assessment of the distribution of measurement values (by determining the confidence intervals) across a range of body sizes in the normal population. The Z score of a measurement is the number of standard deviations of that value from the mean value at a particular BSA. In other words, a Z score of zero corresponds to a measurement equal to the population mean for that particular BSA. A Z score of +2 or −2 corresponds to a measurement that is 2 standard deviations above or below the mean for that particular BSA, thresholds that usually represent the upper or lower limits of normal. Z scores can be converted to percentiles, though the magnitude of an abnormality is much easier to appreciate with Z scores than with percentiles (for example, a Z score of +4 corresponds to the 99.8th percentile, and Z score of +10 corresponds to the 99.9th percentile). The major advantage of using Z scores has been the absence of any reliance on a predetermined relationship between the size of a structure and BSA. In addition, there is no assumption that a constant variance exists across the range of body sizes within the pediatric population. However, the utility of some published Z -score approaches has been limited by the fact that a “normal” population is frequently identified as those individuals with normal results on echocardiography, a truly self-referential definition. Second, the approach does not always account for such confounders as gender and race. Last, the methodologies for performing measurements and calculating BSA are often inconsistent across the entire population being evaluated.
Recommendations: When normative data are available, the measurements of cardiovascular structures should be expressed as Z scores using the Haycock formula to calculate BSA.
Pediatric Quantification Protocols
Pulmonary Veins, Systemic Veins, and Atria
Morphometric Evaluation
The pulmonary veins are usually best evaluated in a high left parasternal or suprasternal short-axis view (“crab” view) displaying drainage of the right and left pulmonary veins into the left atrium. Dual display without and with low-scale color mapping helps identify the individual pulmonary veins, and the diameter of each pulmonary vein before it connects to the left atrium can be measured from the 2D image. The left atrial (LA) appendage should not be mistakenly identified as the left upper pulmonary vein, and the right middle pulmonary vein should not be mistakenly identified as the right upper pulmonary vein (subxiphoid short-axis or right parasternal views are usually better at displaying the right upper pulmonary vein). The superior vena cava is not routinely measured in clinical practice, and normative data have not been established. IVC size can be measured above its junction with the hepatic veins just below the diaphragm in subxiphoid short-axis views (displaying the IVC long axis), and this may help assess hydration status. The IVC diameter varies with respiration, and the collapsibility index (the percentage decrease in IVC diameter with inspiration) appears to correlate with right atrial (RA) pressures in adults. Neither the IVC diameter nor the collapsibility index, however, appears to correlate with age or BSA in adult patients, and the utility of the collapsibility index in children has not been evaluated.
LA size can be assessed by M-mode and 2D measurements of the distance from the posterior aortic wall to the posterior LA wall, though this anteroposterior distance correlates poorly with angiographically derived LA volumes. Recent recommendations involve the calculation of LA volumes from apical views at end-systole just before the MV opens using major-axis and minor-axis dimensions and planimetered areas in orthogonal views ( Figure 1 ). As with all measurements performed in apical views, care must be taken not to foreshorten the heart. Among all LA volume calculations, the biplane area-length and the biplane Simpson (summation of disks) methods using apical 4-chamber and 2-chamber views appear to provide the most consistent results with published reference values for normal adults. The biplane area-length method has also been used in children, and LA volumes indexed to BSA appear to correlate with diastolic function and mitral regurgitation grade. Last, LA volumes have been calculated using real-time three-dimensional (3D) echocardiography and a rotation/polyhedral surface algorithm, and these have correlated well with LA volumes measured by magnetic resonance imaging (MRI).
RA size is usually assessed in an apical 4-chamber view at end-systole just before the TV opens ( Figure 2 ). Major-axis and minor-axis dimensions are significantly different between normal adults and patients with right ventricular (RV) volume overload (from an atrial septal defect or tricuspid regurgitation). Raw values and indexed values on the basis of BSA in normal adults are available. Planimetered RA areas or RA volumes calculated from the product of RA area and major-axis length may be better at assessing RA size, though the sample sizes in most studies have been small. As with LA volumes, 3D echocardiography may provide a useful means by which to measure RA volumes using the polyhedral surface algorithm.
Recommendations ( Table 2 ) : The recommended methods to assess LA size include the measurement of major-axis lengths in apical 4-chamber views and planimetered areas in orthogonal apical views and calculation of volumes using the biplane area-length or the biplane Simpson method. The recommended methods to assess RA size include the measurement of major-axis and minor-axis lengths and planimetered areas in apical 4-chamber views. When the IVC diameter is measured, measurement should be performed above its junction with the hepatic veins just below the diaphragm in subxiphoid short-axis views.
Measurement | View | Timing/Location | Applications | Strengths | Weaknesses |
---|---|---|---|---|---|
LA major-axis length | Apical 4-chamber | Ventricular end-systole ∗ | LA size | Better than M-mode or 2D antero-posterior length Normal adult data Some normal pediatric data | Foreshortening Little normal pediatric data |
LA minor-axis length | Apical 4-chamber | Ventricular end-systole ∗ | LA size | Same as above | Same as above |
LA planimetered area 4C | Apical 4-chamber | Ventricular end-systole ∗ | LA size | Same as above | Same as above |
LA planimetered area 2C | Apical 2-chamber | Ventricular end-systole ∗ | LA size | Same as above | Same as above |
RA major-axis length | Apical 4-chamber | Ventricular end-systole ∗ | RA size | Normal adult data | Same as above |
RA minor-axis length | Apical 4-chamber | Ventricular end-systole ∗ | RA size | Normal adult data | Same as above |
RA planimetered area | Apical 4-chamber | Ventricular end-systole ∗ | RA size | Better than 2D length | Same as above |
IVC diameter | Subxiphoid short-axis | Just below diaphragm | Hydration status RA pressure | Respiratory variation | No normal pediatric data |
Pulmonary vein S velocity | Apical or parasternal short-axis | Systole | LV diastolic function LA function MV function | Depends on alignment | |
Pulmonary vein D velocity | Apical or parasternal short | Diastole | Same as above | Depends on alignment | |
Pulmonary vein Ar velocity | Apical or parasternal short | Diastole | Same as above | Depends on alignment | |
Pulmonary vein Ar duration | Apical or parasternal short | Diastole | Same as above | Depends on alignment |
Calculation | View | Formula | Applications | Strengths | Weaknesses |
---|---|---|---|---|---|
LA volume | Apical | Area-length: 8 × A 4 C × A 2 C 3 × π × h | LA size | Normal adult data | Foreshortening No normal pediatric data |
LA volume | Apical | Summation of disks: π 4 × ∑ i = 1 N a i × b i × h N | LA size | Normal adult data | Foreshortening No normal pediatric data |
Doppler Evaluation
Pulsed-wave Doppler interrogation of pulmonary and systemic venous flow requires precise placement of the sample volume in the lumen of the vessel >5 mm from its ostium. Because venous flow velocities are low, the Doppler high-pass filter should be minimized. Pulmonary venous flow is usually evaluated in apical or parasternal views, whereas superior vena cava flow can be evaluated in subxiphoid or suprasternal views. IVC flow is best evaluated in subxiphoid views; hepatic vein flow is usually used as a surrogate because the hepatic veins are more parallel to the line of interrogation than the IVC. Characterization of pulmonary and systemic venous Doppler patterns can help with the assessment of atrial and ventricular diastolic function as well as AV valve function ( Figure 3 ). Antegrade flow during ventricular systole (S wave) occurs because of both atrial relaxation and apically directed movement of the AV annulus. Occasionally, it is biphasic secondary to temporal dissociation of the two components. Abnormal retrograde flow during ventricular systole can occur in the setting of significant AV valve regurgitation as well as AV electrical dissociation with atrial contraction against a closed AV valve during ventricular systole. Antegrade flow during ventricular diastole (D wave) is influenced by atrial and ventricular filling properties as well as AV valve patency. With fast heart rates, the S and D peaks may fuse. Retrograde flow during atrial contraction (Ar wave) is frequently augmented when ventricular compliance is decreased. In fact, a pulmonary vein Ar wave duration that exceeds the MV inflow duration during atrial systole predicts increased LA and LV end-diastolic pressures in the setting of reduced ventricular compliance. Both the systemic venous D and Ar waves can be affected by respiration, with increased D-wave and decreased Ar-wave velocities during inspiration secondary to negative intrathoracic pressure, so these measurements should be averaged over 3 consecutive cycles.
Recommendations ( Table 2 ) : Pulmonary venous S-wave, D-wave, and Ar-wave velocities and Ar-wave duration are best measured in apical or parasternal short-axis views.
AV Valves
Morphometric Evaluation
Measurement of MV and TV size helps characterize valvar pathology and diagnose ventricular hypoplasia. Annular diameter and area can be measured by 2D and 3D imaging. Annular area may also be estimated from a single-plane diameter using the area formula for a circle, but the MV annulus is in fact elliptical and saddle-like in shape. A more accurate estimate can be obtained with orthogonal diameters using the area formula for an ellipse ([π/4 × diameter 1 × diameter 2 ]). Echocardiographic measurements of MV size generally overestimate postmortem measurements, but this may be an artifact of tissue fixation. Published pediatric normative databases have used annular diameters measured in apical 4-chamber (lateral diameter) and parasternal long-axis (anteroposterior diameter) views to calculate MV and TV elliptical annular areas ( Figure 4 ). However, recent studies in adult patients suggest that apical 2-chamber and 3-chamber views of the MV provide better anatomic alignment and more accurate MV annular dimensions compared with measurements obtained by computed tomography. The difficulty of obtaining adequate 2-chamber views in children often precludes the use of this technique, and most pediatric studies involving various CHDs are based on MV and TV annular measurements obtained in apical 4-chamber and parasternal long-axis views.
The largest diameters during peak filling in early diastole should be measured at the frame after maximum excursion of the leaflets from inner edge to inner edge at the hinge points of the leaflet attachments. Nomograms for MV and TV diameters are available. Although 2D planimetry has been demonstrated to be reasonably accurate in adults with acquired MV stenosis, it is unreliable in the setting of congenital MV stenosis, which is characterized by complex multilevel obstruction with abnormally shaped and often multiple flow orifices precluding a true single-plane “en face” view of the maximum orifice area. Compared with 3D planimetric assessment in patients with mitral stenosis, 2D planimetry overestimates MV area by as much as 88%, depending on valve geometry (domed vs funnel shape) and the position of the transducer relative to the valve orifice.
Recommendations ( Table 3 ) : The recommended methods to assess MV and TV annular size include measurement of lateral diameters in apical 4-chamber views and anteroposterior diameters in parasternal long-axis views and calculation of areas using the area formula for an ellipse.
Measurement | View | Timing | Applications | Strengths | Weaknesses |
---|---|---|---|---|---|
Lateral MV diameter (MVD L ) | Apical 4-chamber | Diastole ∗ | MV size | Reproducible Normal adult data Normal pediatric data | May not be as good as apical 2-chamber and apical 3-chamber measurements |
Antero-posterior MV diameter (MVD AP ) | Parasternal long-axis | Diastole ∗ | MV size | Same as above | Same as above |
Lateral TV diameter (TVD L ) | Apical 4-chamber | Diastole ∗ | TV size | Same as above | |
Antero-posterior TV diameter (TVD AP ) | Parasternal long-axis | Diastole ∗ | TV size | Same as above | |
MV E wave peak velocity | Apical 4-chamber | Diastole | LV diastolic function | Depends on alignment & sample volume location Depends on loading conditions | |
MV A wave peak velocity | Apical 4 | Diastole | LV diastolic function | Same as above | |
MV A wave duration | Apical 4 | Time from beginning to end of A wave | LV diastolic function | Fast heart rates in children → fusion of E and A waves | |
MV deceleration time | Apical 4 | Time from E wave peak velocity to return to baseline | LV diastolic function | Fast heart rates in children → fusion of E and A waves | |
Isovolumic relaxation time (IVRT) † | Apical 3-chamber | Time from AoV closure to MV opening with simultaneous CW Doppler of LV outflow and inflow | LV diastolic function | Fast heart rates in children → poor temporal resolution |
Calculation | View | Formula | Applications | Strengths | Weaknesses |
---|---|---|---|---|---|
MV area | Apical 4-chamber/parasternal long-axis | π 4 × M V D L × M V D A P | MV size | Reproducible Normal adult data Normal pediatric data | Assumes elliptical shape Measures annular area and not valvar area |
TV area | Apical 4-chamber/parasternal long-axis | π 4 × T V D L × T V D A P | TV size | Same as above | Assumes elliptical shape Measures annular area and not valvar area |
E/A ratio | Apical 4-chamber | E wave peak velocity/A wave peak velocity | LV diastolic function | Fast heart rates in children → fusion of E and A waves |
† Measured by blood flow Doppler evaluation rather than by tissue Doppler evaluation, as described in Table 4 .
Doppler Evaluation
Doppler interrogation of ventricular inflow is best performed with the help of color mapping in apical views where transducer position and angulation changes are frequently needed to optimize alignment. When MV or TV stenosis is suspected, the VTI of the inflow tracing from continuous-wave Doppler interrogation is used to calculate the mean gradient and assess the severity of obstruction. It is important to remember, however, that the transvalvar gradient is dependent on the diastolic filling period and can be augmented by the faster heart rates in children. Stenosis can also be evaluated by measuring the pressure half-time (the time needed for the peak early diastolic pressure to decline by 50%) or calculating the effective orifice area from the continuity equation (the stroke volume or the product of cross-sectional area and the blood flow VTI at that location is preserved at each position along a closed system). However, these methods are also limited by the faster heart rates in children, correlate poorly with data derived from catheterization in the setting of congenital AV valve stenosis, and are not recommended for routine use in children. Quantitative assessment of MV and TV regurgitation has been discussed previously for adults. Some of the recommended Doppler methods have included measurement of the vena contracta diameter and the regurgitant jet area as well as calculation of the regurgitant volume, regurgitant fraction, and effective regurgitant orifice area from the continuity equation and from the proximal isovelocity surface area phenomenon. However, the utility of these indices in children is limited, and they have not been validated.
Pulsed-wave Doppler analysis of MV inflow velocities is used frequently to assess LV diastolic function. The sample volume is best positioned in the left ventricle at the tips of the valve leaflets (distal to the annulus) because both the peak early diastolic velocity (E wave) and the peak velocity during atrial contraction (A wave) decrease significantly in value as the sample volume is moved toward the atrium ( Figure 5 A). The isovolumic relaxation time (IVRT), representing the time from AoV closure to MV opening, can be measured from the aortic component of the second heart sound using a phonocardiogram to the onset of diastolic flow in the MV Doppler tracing or by using simultaneous continuous-wave Doppler interrogation of LV inflow and outflow in an apical 3-chamber view ( Figure 5 B). The deceleration time from the peak E-wave velocity to its return to baseline in mid-diastole is another parameter of diastolic function that is sensitive to ventricular relaxation and compliance as well as atrial pressure ( Figure 5 C). However, deceleration time and other diastolic indices based on the E and A waves are limited by their dependence on loading conditions, and their utility in children is often precluded by fusion of the E and A waves resulting from rapid heart rates.
LV diastolic filling can also be characterized by using several calculations : the ratio between the E-wave and A-wave velocities; the E-area and A-area fractions, comparing the VTI during early diastole (E area) and during atrial contraction (A area) with the total area under the diastolic curve; the area or filling fraction in the first 33% (one third filling faction) or the first 50% (one half filling fraction) of diastole; the ratio between pulmonary vein Ar-wave duration and MV A-wave duration as discussed previously ( Figure 5 D); and peak ventricular filling rates from the product of the E velocity and MV annular cross-sectional area. Because filling rates may vary with cardiac output, it may be more useful to calculate peak filling rates normalized to stroke volume (PFR SV ) using the following equation :
However, an important limitation with this approach involves the fact that calculations of LV inflow (flow across the MV annulus) and peak filling rates using pulsed-wave Doppler interrogation do not account for MV annular displacement away from the transducer during diastole; hence, the Doppler profiles used in these calculations actually represent flow toward the transducer rather than true flow across the MV annulus.
Recommendations ( Table 3 ) : The recommended methods to assess MV inflow include measurements of E-wave and A-wave velocities, A-wave duration, deceleration time, and IVRT and calculation of the E/A ratio.
Left Ventricle
Morphometric Evaluation
Measurements of LV size and function are essential in the assessment of patients with congenital and acquired heart diseases. Although qualitative visual inspection might be adequate, it can be misleading, is prone to interobserver and interstudy variability, and relies on the skill of the interpreter. Given the impact of quantitative data on diagnosis and management, the importance of accurate, reproducible LV measurements cannot be overstated. Although published ASE recommendations for chamber quantification in adults have been used extensively in children, data on the accuracy and reproducibility of these measurements in pediatrics are scant. In addition, there are limitations to the published methods adjusting for body size in adults as discussed previously. Several linear and volumetric methods to assess LV size have been described and integrated into routine clinical practice, each with distinct advantages and weaknesses, and these are discussed in this section.
In general, LV size should be measured during both diastole and systole, defining end-diastole as the frame with the maximum chamber intraluminal area and end-systole as the frame with the minimum area. However, these definitions are problematic because they rely on visual estimates of areas rather than a quantitative frame-by-frame analysis. In addition, the minimum area occurs at different times in short-axis and long-axis views. During isovolumic contraction, the long axis first shortens and then elongates (the reverse process occurs during isovolumic relaxation). In contrast, the short-axis area first increases and then decreases progressively during isovolumic contraction. Given these limitations, end-diastole can be defined as the frame at which the MV closes and end-systole as the frame preceding MV opening.
Short-axis or minor-axis measurements of LV internal diameter and septal and posterior wall thickness can be obtained in parasternal views ( Figure 6 ), though occasionally these measurements are available only in subxiphoid views. The maximum short-axis dimension is often located at the level of the MV leaflet tips or chordae in young patients and more apically at the level of the papillary muscles in older patients and some adults. It is important to note that linear measurements characterize LV size only in one dimension and may misrepresent an abnormally shaped chamber. Short-axis diameters should be considered a surrogate for LV size only when the LV short-axis geometry is circular, a condition often not met in patients with CHD or other abnormal hemodynamic states. Linear measurements can be obtained from long-axis or short-axis views and from M-mode tracings or 2D images. The ASE guidelines for adults recommend linear minor-axis measurements of the left ventricle in parasternal long-axis views because this ensures a perpendicular orientation between the measurement and the LV long axis. In addition, limited parasternal windows can overestimate minor-axis diameters with oblique measurements in apically located short-axis views, a problem not seen with long-axis views in nonstandard parasternal locations. However, a long-axis view does not account for the lateral motion of the left ventricle seen in many children and it does not guarantee circular LV short-axis geometry throughout the cardiac cycle. It also forces the use of a single diameter, in contrast to the multiple diameters available from 2D short-axis images. Consequently, the short-axis view is the recommended approach because it allows one to choose the diameter with the best blood-endocardium interface, a definite advantage when dealing with LV trabeculations. In addition, normal pediatric data from M-mode short-axis views are available.
M-mode echocardiography has provided better temporal and spatial resolution than 2D imaging in the past. However, M-mode measurements in long-axis views can overestimate LV minor-axis diameters compared with 2D measurements. In addition, measurements along a line crossing the midpoints of the ventricular septum and posterior wall (on the basis of the location of the papillary muscle groups) can be difficult by M-mode. Refinements in transducer technology and image processing have recently provided 2D imaging with improved resolution and clear delineation of the blood-endocardium interface. Therefore, 2D short-axis imaging is the recommended approach to obtain LV short-axis measurements averaged over 3 consecutive cardiac cycles ( Figure 6 ). Ideally, a combination of long-axis and short-axis views should be used to ascertain that the short-axis or minor-axis measurement is perpendicular to and crosses the midpoints of the ventricular septum and posterior wall and that the LV short-axis geometry is circular throughout the cardiac cycle.
Two-dimensional volumetric methods require quality LV images from parasternal short-axis, apical, and/or subxiphoid views in which the LV major-axis length and area of the LV endocardial border can be measured. The basal border is defined as the line connecting the MV annular hinge points. The LV length is measured from the basal border midpoint to the apical endocardium, requiring clear images of the apical endocardium without foreshortening the left ventricle. The endocardial border is traced manually, requiring clear delineation of the blood-endocardium interface (convention excludes the papillary muscles when tracing the endocardial border, leaving them included in the blood pool). The biplane Simpson method to calculate LV volumes by summation of equidistant disks is used frequently in adults, with few data validating its accuracy and reproducibility in children. It involves tracing the LV endocardial border in apical 4-chamber and 2-chamber views and using the formula
where V is volume, a i is the minor-axis slice radius in the apical 4-chamber view, b i is the minor-axis slice radius in the apical 2-chamber view, L is the LV major-axis length, and N is the number of slices ( Figure 7 ). Some have suggested that the apical 3-chamber view can be substituted for the apical 2-chamber view. In children with abnormally shaped left ventricles, the modified Simpson algorithm using a combination of short-axis and long-axis views may be better than the biapical algorithm described above. LV volume can also be measured with the area-length or bullet method using the formula V = 5/6 × short-axis basal area × LV length ( Figure 8 ). Here, the short-axis basal area is measured from parasternal or subxiphoid short-axis views, and LV length is measured from apical 4-chamber or subxiphoid long-axis views. The truncated ellipse method is similar to the area-length method, with a somewhat different formula requiring the additional measurement of the LV minor-axis diameter from an apical 4-chamber view.
LV mass can be calculated from M-mode or 2D linear measurements, and this approach has been used extensively in adult clinical trials and epidemiologic studies. It has been used in children, though accuracy and reproducibility data, especially in infants, are scant. The most common method is to measure volumes using one of the approaches discussed previously. LV mass is then calculated by subtracting the endocardial volume from the epicardial volume and multiplying this difference (the myocardial volume) by 1.05 g/mL, the myocardial-specific density. LV volume and mass can also be measured using 3D echocardiography, and growing experience suggests that better accuracy can be achieved compared with 2D methods when MRI is used as the gold standard. Initial reports in pediatrics are encouraging, particularly considering that 3D echocardiography does not rely on geometric assumptions, an important advantage in patients with CHD and abnormally shaped ventricles. However, the feasibility, applicability, and reproducibility of this approach in clinical practice warrant further investigation.
LV systolic function can be evaluated as pump function (global chamber performance) or myocardial function (performance of cardiac myofibers). Global systolic pump function is dependent on myocardial force generation characteristics (contractility) as well as preload, afterload, and heart rate, whereas myocardial function represents myocardial contractility independent of loading conditions and heart rate. Numerous echocardiographic methods have been used to evaluate both properties of LV systolic function, and these can be divided into geometric and nongeometric parameters. Geometric parameters require LV dimension or volume measurements and are influenced by LV shape. Nongeometric parameters do not require these measurements, are not affected by LV shape, and rely on Doppler echocardiography and other techniques.
The most commonly used geometric parameters of global LV systolic function are the linear shortening fraction (SF), fractional area change, and the volumetric ejection fraction (EF). These methods are affected by loading conditions, but fractional area change and EF are less sensitive to abnormal chamber geometry and regional abnormalities. SF can be calculated using LV minor-axis internal diameters obtained from a standard M-mode tracing or from 2D images using the equation SF = (end-diastolic dimension − end-systolic dimension)/end-diastolic dimension. As discussed previously, the 2D approach provides better display of the midpoints of the ventricular septum and posterior wall. EF is calculated from the equation EF = (end-diastolic volume − end-systolic volume)/end-diastolic volume; both end-diastolic and end-systolic volumes are measured using any of the 2D or 3D methods described previously. Extrapolation of EF from linear LV minor-axis diameters is discouraged, because of inaccuracies resulting from geometric assumptions. A relatively load independent index of myocardial function is useful when following patients at risk for abnormal afterload, such as patients undergoing chemotherapy and those infected with human immunodeficiency virus. One such method involves the relationship between velocity of circumferential fiber shortening adjusted for heart rate and end-systolic wall stress, and this index of contractility is relatively independent of preload and incorporates afterload and heart rate in its assessment ( Figure 9 ). However, this index applies only when the LV is normally shaped.
Recommendations ( Table 4 ) : The recommended methods to assess LV size and function include a linear approach and a volumetric approach. The linear method involves measurement of short-axis diameters and wall thickness and calculation of SF and the velocity of circumferential fiber shortening adjusted for heart rate and end-systolic wall stress from 2D short-axis images obtained in parasternal or subxiphoid short-axis views. The volumetric method involves (1) measurement of areas from the same 2D or 3D short-axis images; (2) measurement of long-axis lengths from 2D or 3D long-axis images obtained in apical 4-chamber or subxiphoid long-axis views; and (3) calculation of volumes, EF, and mass using 2D or 3D measurements.
Measurement | Technique/View | Timing/Location | Applications | Strengths | Weaknesses |
---|---|---|---|---|---|
End-diastolic diameter (EDD) | 2D Parasternal or subxiphoid short-axis | End-diastole ∗ just below mitral annulus | LV size | Adjustable alignment Normal adult data Normal pediatric data | Lower temporal resolution than M-mode Inappropriate for abnormal LV shape Depends on good blood-endocardium border No normal data |
End-diastolic posterior wall thickness (EDPWT) | 2D Parasternal or subxiphoid short-axis | End-diastole ∗ just below mitral annulus | LV size | Adjustable alignment Normal adult data Normal pediatric data | Same as above |
End-diastolic septal wall thickness (EDSWT) | 2D Parasternal or subxiphoid short-axis | End-diastole ∗ just below mitral annulus | LV size | Adjustable alignment Normal adult data Normal pediatric data | Same as above |
End-systolic diameter (ESD) | 2D Parasternal or subxiphoid short-axis | End-systole † just below mitral annulus | LV size | Adjustable alignment Normal adult data Normal pediatric data | Same as above |
End-systolic posterior wall thickness (ESPWT) | 2D Parasternal or subxiphoid short-axis | End-systole † just below mitral annulus | LV size | Adjustable alignment Normal adult data Normal pediatric data | Same as above |
End-systolic septal wall thickness (ESSWT) | 2D Parasternal or subxiphoid short-axis | End-systole † just below mitral annulus | LV size | Adjustable alignment Normal adult data Normal pediatric data | Same as above |
End-diastolic length (EDL) | 2D Apical 4-chamber or subxiphoid long-axis | End-diastole ∗ | LV size | Adjustable alignment Normal adult data Normal pediatric data | Foreshortening Depends on distinct apical endocardium |
End-diastolic epicardial length (EDL epi ) | 2D Apical 4-chamber or subxiphoid long-axis | End-diastole ∗ | LV size | Adjustable alignment Normal adult data Normal pediatric data | Foreshortening Depends on distinct apical endocardium |
End-diastolic area (EDA) | 2D Parasternal or subxiphoid short-axis | End-diastole ∗ | LV size | Adjustable alignment Normal adult data Normal pediatric data | Depends on good blood-endocardium border |
End-diastolic epicardial area (EDA epi ) | 2D Parasternal or subxiphoid short-axis | End-diastole ∗ | LV size | Adjustable alignment Normal adult data Normal pediatric data | Depends on good blood-endocardium border |
End-systolic length (ESL) | 2D Apical 4-chamber or subxiphoid long-axis | End-systole ∗ | LV size | Adjustable alignment Normal adult data Normal pediatric data | Foreshortening Depends on distinct apical endocardium |
End-systolic epicardial length (ESL epi ) | 2D Apical 4-chamber or subxiphoid long-axis | End-systole ∗ | LV size | Adjustable alignment Normal adult data Normal pediatric data | Foreshortening Depends on distinct apical endocardium |
End-systolic area (ESA) | 2D Parasternal or subxiphoid short-axis | End-systole ∗ | LV size | Adjustable alignment Normal adult data Normal pediatric data | Depends on good blood-endocardium border |
End-systolic epicardial area (ESA epi ) | 2D Parasternal or subxiphoid short-axis | End-systole † | LV size | Adjustable alignment Normal adult data Normal pediatric data | Depends on good blood-endocardium border |
Peak MV annular velocity in early diastole (e’) | Tissue Doppler Apical 4-chamber | Early diastole at lateral & medial MV annulus | LV diastolic function | Reproducible Good temporal resolution Normal pediatric data | Depends on alignment (angle-dependent) Depends on loading conditions Not useful for regional wall motion abnormalities |
Peak MV annular velocity at atrial contraction (a’) | Tissue Doppler Apical 4-chamber | Atrial contraction at lateral & medial MV annulus | LV diastolic function | Same as above | Same as above |
Peak MV annular velocity in systole (s’) | Tissue Doppler Apical 4-chamber | Systole at lateral & medial MV annulus | LV systolic function | Same as above | Same as above |
Peak MV annular velocity in isovolumic contraction | Tissue Doppler Apical 4-chamber | Isovolumic contraction at lateral & medial MV annulus | LV systolic function | Same as above | Same as above |
Isovolumic relaxation time (IVRT’) ‡ | Tissue Doppler Apical 4-chamber | Time from end of s’ wave to beginning of e’ wave | LV diastolic function | Same as above | Same as above |
Isovolumic contraction time (IVCT’) | Tissue Doppler Apical 4-chamber | Time from end of a’ wave to beginning of s’ wave | LV systolic function | Same as above | Same as above |
Time to peak velocity in isovolumic contraction | Tissue Doppler Apical 4-chamber | Isovolumic contraction at lateral & medial MV annulus | LV systolic function | Same as above | Same as above |
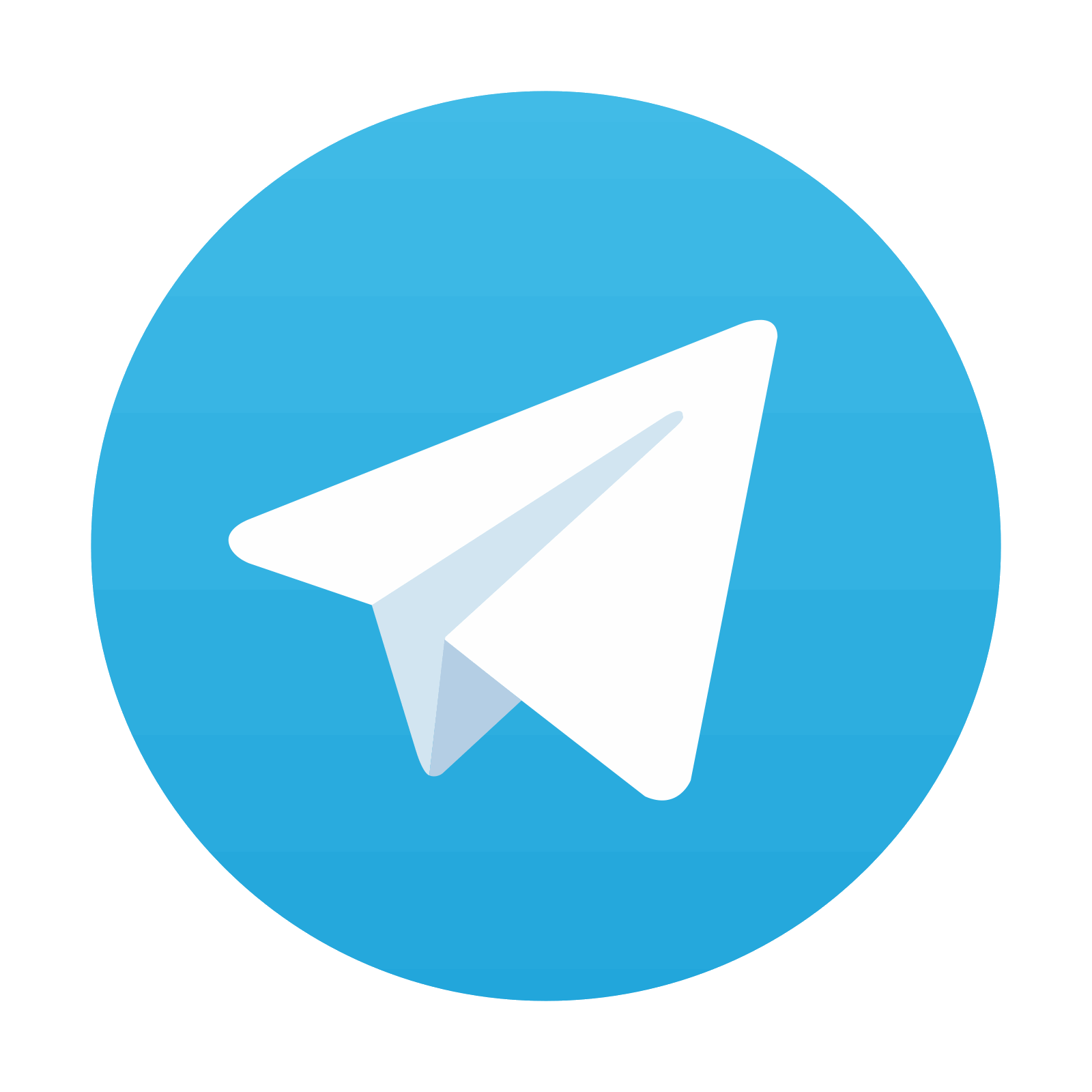
Stay updated, free articles. Join our Telegram channel
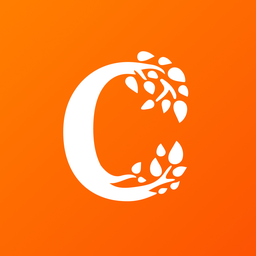
Full access? Get Clinical Tree
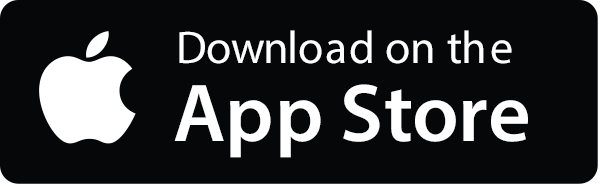
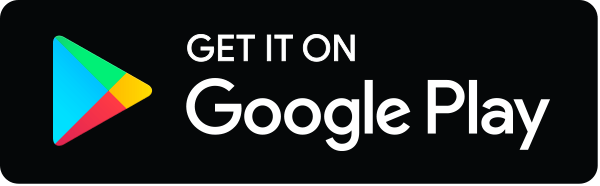
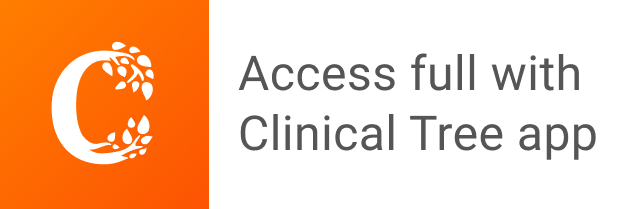