It is recognized by many in the field of nuclear cardiology that in order to thrive and advance, the discipline needs to go beyond myocardial perfusion imaging (MPI). The high diagnostic and risk stratification utilities of radionuclide single-photon emission computed tomography (SPECT) and positron emission tomography (PET) MPI are well established,1–4 with observational studies5–7 strongly suggesting that performing and properly acting upon the results of MPI can lead to improved patient outcome, with a study in progress designed to firmly establish this.8 Nevertheless, there is an increased focus on developing radionuclide techniques that rely on a unique strength of the modality, that is, the ability to image the underlying molecular processes of cardiac disease.9 Among such nonperfusion radionuclide imaging methods of current interest is assessment of cardiac autonomic innervation.10,11
Neurohormonal regulation of the cardiovascular system is crucial for maintaining body function. One major component is circulating hormones that include epinephrine, norepinephrine (NE), arginine vasopressin, B-type natriuretic peptide (BNP), and substances of the renin-angiotensin aldosterone system (RAAS).12 Another component, working in conjunction with the hormonal mediators, is direct innervation via the sympathetic and parasympathetic autonomic nervous system. Together, these neurohormonal (sometimes also referred to as “neurohumoral”) processes control cardiac output (CO), vascular tone, and blood volume, serving to maintain proper perfusion to body organs as needed for normal conditions and activities. In response to stressors or insults, such as volume depletion or diminished CO from cardiac dysfunction, neurohormonal mechanisms compensate with vasoconstriction, augmentation of myocardial contractility, increased heart rate, and expansion of extracellular volume.13 In many situations, such adaptations are necessary and beneficial, but in other circumstances neurohormonal activation can be excessive and persist beyond what is needed, thus maladaptive and potentially harmful.14 The ability to directly visualize these mechanisms via radionuclide imaging provides important insights into the pathophysiology of various cardiac diseases. Much recent work shows a robust ability of cardiac autonomic innervation imaging to effectively assess a patient’s condition beyond other commonly used methods. There is much promise that such imaging will lead to improved disease management, thus a reduction of adverse events and improved patient outcome and well-being.
The heart is richly innervated by sympathetic and parasympathetic fibers which are controlled by regulatory centers in the brain that integrate input signals from other parts of the brain and from receptors throughout the body. Efferent sympathetic signals from the regulatory centers follow descending pathways in the spinal cord, and synapse with preganglionic fibers that leave the spinal cord at levels T1–L3, subsequently synapsing with paravertebral stellate ganglia, and eventually innervating the right ventricle, and the anterior and lateral left ventricle. In the heart sympathetic nerves follow the coronary arteries in the subepicardium, and then penetrate the myocardium. Adrenergic output from this sympathetic innervation increases HR (chronotropic effect), augments contractility (inotropic effect), and enhances atrioventricular (AV) conduction (dromotropic effect). The major chemical mediator of sympathetic innervation is NE.15,16
Parasympathetic fibers, scarce in comparison with sympathetic fibers, originate in the medulla and travel alongside the vagus nerves. In the heart, these fibers start epicardially, cross the AV groove, and penetrate the myocardium, thereby becoming located in the subendocardium. Parasympathetic fibers predominantly innervate the atria, but are scarce in the ventricle (densest in the inferior wall). The parasympathetic system modulates output of the sinoatrial (SA) and AV nodes to control HR and conduction. The chemical mediator of parasympathetic function is acetylcholine.
Most published literature and impending clinical applicability of autonomic radionuclide imaging is of the sympathetic system, with parasympathetic imaging limited mostly to animals. The ensuing discussion will focus on cardiac sympathetic imaging.
Cardiac sympathetic innervation imaging visualizes processes at the synaptic junction, illustrated in Figure 23-1.17 To date, most autonomic tracers investigated image presynaptic anatomy and function, though tracers designed to specifically assess postsynaptic α- and β-receptors have also been studied.
Figure 23-1
Schematic representation of the sympathetic neuron synapse. In response to a stimulus, vesicles containing NE are released into the synaptic space and interact with postsynaptic receptors. Sympathetic response is controlled by reuptake of norepinephrine into the presynaptic terminal via the “uptake-1” norepinephrine transporter. AC, adenylyl cyclase; AMP, adenosine monophosphate; cAMP, cyclic adenosine monophosphate; G, G proteins; NE, norepinephrine. (Reproduced with permission from Travin MI. Cardiac neuronal imaging at the edge of clinical application. Cardiol Clin. 2009;27(2):311–327.)

NE is synthesized in presynaptic sympathetic terminals from tyrosine, and stored in presynaptic vesicles. In response to stimuli, the vesicles are released into the synaptic space, freeing the NE which interacts with postsynaptic α, β1, and β2 receptors on the myocyte membrane, leading to adrenergic effect(s).18,19 To control and terminate these effects, NE is actively taken back into the presynaptic terminal mostly via norepinephrine transporter-1 (NET1), a protein-mediated, sodium-, energy-, and temperature-dependent “uptake-1” process, for storage or catabolic disposal by monoamine oxidase (MAO) or catechol-o-methyltransferase.20 A smaller amount of NE is also taken up by postsynaptic, nonneuronal cells, probably by sodium-independent passive diffusion, referred to as the “uptake-2” process.21,22
In the 1970s, while investigating adrenal imaging using modifications of the adrenergic blocking antiarrhythmic drug bretylium, Wieland et al. focused on a similar false neurotransmitter analogue of NE, guanethidine, and discovered that synthesizing a compound with iodine in the meta position, that is, meta-iodobenzylguanidine (mIBG) could satisfactorily image both the adrenal medulla and the heart.23–25 However, unlike NE, this false neurotransmitter is not catabolized after NET1 uptake, thus localizing to high concentrations in the presynaptic adrenergic terminals. Following IV injection of iodine-labeled radiotracer that diffuses into cardiac synaptic clefts and is taken via NET1 into cardiac presympathetic terminals, the heart can be visualized, with the measured counts reflecting the physical integrity and physiologic health of cardiac adrenergic innervation.26,27 The first cardiac mIBG images in humans are shown in Figure 23-2. As opposed to 201Tl- or 99mTc-based perfusion tracers that image the “plumbing of the heart,” mIBG has been characterized as providing “clinicians with insight into ‘wiring’ of the heart.”23
Figure 23-2
The first images showing cardiac uptake of 123I-mIBG in humans. 123I-mIBG images of myocardium in five normal volunteers, acquired over 15 minutes in the 40-degree angle LAO projection. (A) Unprocessed digital images. (B) Images after interpolated background subtraction. LAO, left anterior oblique; mIBG, metaiodobenzylguanidine. (Reproduced with permission from Kline RC, Swanson DP, Wieland DM, et al. Myocardial imaging in man with I-123 meta-iodobenzylguanidine. J Nucl Med. 1981;22(2):129–132.)

Initial clinical work that focused on adrenal tumor imaging used iodine-131 for labeling, but as this isotope gives off relatively high-energy (365 keV) γ emissions, emits β particles, and has a relatively long half-life of about 8.02 days, 123I labeling has been developed and is preferred. 123I emits predominantly γ photons with energies of 159 keV and has a half-life of 13.2 hours, therefore easily imaged and well tolerated. Thus, 123I meta-iodobenzylguanidine (123I-mIBG) is currently the preferred compound for clinical use, and was approved by the Food and Drug Administration (FDA) for cardiac use in 2013.
Various PET adrenergic radiotracers have also been developed, but are currently under investigation and not approved for clinical use.22 Compared with 123I-mIBG, PET tracers not only have superior physical properties for imaging, but also potential biologic advantages. 11C-meta-hydroxyephedrine (HED) has been most widely studied, with its higher NET1 selectivity resulting in better differentiation between innervated and denervated myocardium, reportedly better for evaluating neuronal heterogeneity in hibernating myocardium.28 Other less well-studied PET tracers include 11C-epinephrine and 11C-phenylephrine. However, as the need for a nearby cyclotron makes 11C-tracers impractical for most potential users, 18F compounds, such as 18F-LMI-1195 (fluorobenzylguanidine) are in development.29
123I-mIBG is performed at rest and requires only minimal preparation, with details described in published guidelines.30,31 The patient is customarily kept NPO after midnight on the day of imaging. Standard guidelines directed HF medications, such as β blockers, angiotensin-converting enzyme inhibitors (ACE-I), and/or angiotensin receptor blockers (ARBs), need not be withheld. Published guidelines list and recommend holding medications and substances known to interfere directly with the mechanisms of NE uptake and granule storage, such as opioids, cocaine, tramadol, tricyclic antidepressants, sympathomimetics and antipsychotics, as well as foods containing vanillin (an artificial substance added as a flavoring agent to various foods, beverages, and pharmaceuticals) and catecholamine-like compounds (e.g., chocolate and blue cheese).30–33 There is a report that neuropsychiatric medications with high potency can result in a falsely decreased tracer uptake in the heart and thus overestimate cardiac risk.34 Regarding standard cardiovascular and antihypertensive medications, other than labetalol and rarely used reserpine, there is no solid evidence that β blockers or calcium channel blockers directly affect cardiac uptake. Investigations into this matter are hampered by the fact that cardiovascular medicines can alter cardiac 123I-mIBG uptake by improving a patient’s cardiac condition as opposed to having a direct effect on cellular mechanisms.35
Recommendations regarding medication holding prior to an 123I-mIBG study remain under investigation. At the time of this writing, the specific wording in the package insert from a commercially available preparation is to “consider the withdrawal of the following categories of medications if the withdrawal can be accomplished safely: antihypertensives that deplete NE stores or inhibit reuptake (e.g., reserpine, labetalol), antidepressants that inhibit NE transporter function (e.g., amitriptyline and derivatives, imipramine and derivatives, selective serotonin reuptake inhibitors), and sympathomimetic amines (e.g., phenylephrine, phenylpropanolamine, pseudoephedrine, and ephedrine). The period of time necessary to discontinue any specific medication prior to 123I-mIBG dosing has not been established.”36
Opinions differ with respect to the need for prophylactic administration of iodine to block thyroid uptake of unbound radioactive 123I. While some people advocate routine pretreatment to prevent thyroid radiation exposure from the unbound impurities, others feel that the risk of iodine allergy outweighs the danger from the minimal amount of unbound tracer present after modern production methods. Nevertheless, one study showed that even with an 123I-mIBG preparation of 98% radiochemical purity, unblocked patients have a 50% higher 4-hour thyroid accumulation of unbound 123I compared with pretreated patients, with an estimated 70-mGy exposure from a 10-mCi dose.37 One approach is that pretreatment can be individualized, with greater consideration given to pretreatment for younger patients, but less for elderly patients with multiple comorbidities and potential risk of iodine allergy.38
If chosen, thyroid blockade can be achieved with oral administration of solutions of potassium iodide or Lugol’s (100-mg iodide for adults, body weight adjusted for children), or for patients allergic to iodine, potassium perchlorate (400 mg for adults, weight adjusted for children), orally administered at least 1 hour, minutes prior to mIBG injection.36
At the time of this writing, the specific wording in the package insert from a commercially available preparation is that thyroid blockade should be done in “patients at risk for thyroid accumulation of the drug,” but can be individualized in that “blockade may not be necessary for patients who have undergone thyroidectomy or those with a very limited life expectancy.” The package insert also states that “failure to block thyroid uptake of 123I may result in an increased long-term risk for thyroid neoplasia.”36
Regarding tracer dosage, older studies in the literature used 3 to 5 mCi (111–185 MBq). However, as these dosages frequently result in unsatisfactory SPECT images, particularly in patients with severe cardiac dysfunction, recent investigations have used up to 10 mCi (370 MBq)18 including a phase III study39,40 that helped lead to FDA approval. A 10-mCi dosage results in a radiation exposure of ~5 mSv, with the highest exposure to the bladder, liver, spleen, gall bladder, heart, and adrenals; the absorbed dose may be higher in patients with severe renal impairment.30 Recent work suggests that with a solid-state camera, satisfactory planar and tomographic images can be obtained with a reduced tracer dosage of 2 to 3 mCi (74–111 MBq).41
It has been recommended that patients lie quietly in a supine position for about 5 to 15 minutes before administration. As initial images are acquired a few minutes after injection, the patient should be lying under the camera or in close proximity. Tracer is then administered slowly over 1 to 2 minutes, followed by a saline flush. Adverse reactions are extremely rare.
While acquisition and processing of planar and SPECT 123I-mIBG images are customarily performed in a manner typical for perfusion imaging, special issues need to be considered, and there will likely be methodological changes in the future. The current key parameters for 123I-mIBG image interpretation—heart-to-mediastinum ratio (HMR) and washout—require accurate and robust quantitative analysis of counts in the heart and adjacent background regions, and thus could vary in relation to differences in the acquisition field of view, the type of collimator used, and how the multiple energy emissions of 123I are considered during acquisition and processing. Current literature data are based on images obtained without attenuation correction, Compton scatter, or depth-dependent loss of spatial resolution, with use of such techniques expected to produce different values. Finally, increased use of solid-state cameras results in quantitative 123I-mIBG results from what has been reported for standard Anger cameras.
Figure 23-3 shows a guidelines-recommended protocol for performing cardiac 123I-mIBG imaging.31 Anterior planar 123I-mIBG images are acquired for 10 minutes with the patient supine at ~15 minutes (early) and beginning ~3 hours, 50 minutes (late) after tracer administration, with the images stored in a 128 × 128 or 256 × 256 matrix.30,38 The field of view should include as much of the heart and upper chest as possible, avoiding having the heart be too close to the edge of the field or too close to the center. Positioning should be the same for the early and late planar images.
Figure 23-3
A recommended protocol for performing cardiac 123I-mIBG imaging. Ant, anterior; KI, potassium iodide; MBq, megabecquerel; mCi, millicurie; mIBG, meta-iodobenzylguanidine; SPECT, single-photon emission computed tomography. (Reproduced with permission from Henzlova MJ, Duvall WL, Einstein AJ, et al. ASNC imaging guidelines for SPECT nuclear cardiology procedures: stress, protocols, and tracers. J Nucl Cardiol. 2016;23(3):606–639.)

A large field-of-view camera has customarily been used for acquiring the anterior planar image that is important for deriving global cardiac uptake as measured by the HMR. The technique for obtaining suitable images from a small-field-of-view SPECT camera is unclear. In a pilot study of 67 subjects, HMRs calculated from SPECT images, using mean counts between heart and mediastinum volumes of interest drawn on transaxial images, were equivalent to those obtained by planar techniques for differentiating subjects with normal versus abnormal mIBG uptake.42 More investigations are required to validate this further. Investigations are also ongoing to determine proper techniques for acquiring and interpreting images with solid-state cameras.43
Although the utility of SPECT imaging assessment of regional defects is currently uncertain, and thus considered in guidelines as an option,30 based on theoretic consideration44 and reports showing utility for arrhythmias,45–47 tomographic acquisitions are commonly performed immediately following both the early and late planar images. SPECT images are obtained using a standard 180-degree angle circular acquisition from 45-degree angle right anterior oblique to 45-degree angle left posterior oblique, with a total of 60 stops (30 stops per head if done with a dual-headed camera) at 30 seconds per stop, and are stored in a 64 × 64 matrix.
Extensive literature describes data derived from image acquisition using low-energy high-resolution (LEHR) collimators, with a symmetrically centered energy window of 20% around the main 159-keV isotope photopeak. However, HMR values vary depending on the collimator used, differing for low-energy versus medium-energy collimators, but also among different collimator brands.48–50 Contributing to these differences are multiple higher-energy photon emissions (e.g., 529 keV) that penetrate the septa and degrade image quality.51 Methods to compensate for this effect and improve quantitative accuracy are under investigation, including employing a calibration phantom to derive a conversion coefficient for each camera-collimation system.52–54
Protocols for acquiring and processing 123I-mIBG images will evolve with increased clinical use and further investigations. Ultimately, the standard will depend on the technique that provides the best clinical value in relation to the lowest radiation exposure, and to the highest efficiency and patient comfort.
Most published studies of 123I-mIBG have focused on planar imaging. The standard measure of global tracer uptake is the HMR, derived from regions of interest (ROIs) over the heart and upper mediastinum in an anterior planar image. Uptake in late 123I-mIBG images is considered more representative of tracer retention, and is better predictive of patient outcome than that in early images.40 While various methods have been used to create ROIs,55–57 the currently recommended method is dividing counts per pixel in an irregular ROI defining the epicardial border of the heart by counts per pixel in a square 7 × 7 pixel ROI in the upper mediastinum.30,31 While there are attempts to automate the process,58 at present the standard is manual drawing of ROIs that has been shown to be robust,59 with Veltman et al.60 reporting extremely high intraclass correlation coefficients 0.98 and 0.96 for intra- and interobserver analyses, respectively. Normal HMRs (for Anger cameras) range from 1.9 to 2.8, a mean of 2.2 ± 0.3 with ≥1.6 (within 2 standard deviations) on the late image indicating lower risk.26,39,40 Recent work suggests that because of differences in scatter, HMRs derived from solid-state camera-acquired images are higher.43 Figure 23-4 shows representative planar images with HMRs from patients in different stages of heart failure (HF).61
Figure 23-4
Examples of planar 123I-mIBG images in patients with heart failure, with HMRs and patient outcomes described. (A) A 79-year-old male with nonischemic NYHA II HF and LVEF of 35%. HMR = 2.02. No cardiac event over 5-year follow-up. (B) A 64-year-old female with nonischemic NYHA III HF and LVEF 25% to 30%. HMR = 1.38. Experienced numerous arrhythmic events/ICD discharges over the next 2 years, requiring VT ablation. (C) A 28-year-old male with nonischemic NYHA II HF and LVEF = 20%. HMR = 1.17 (cardiac uptake is barely above background). Progressed within 1 year from NYHA II to NYHA III. HF, heart failure; HMR, heart-to-mediastinum ratio; ICD, implantable cardioverter defibrillator; LVEF, left ventricular ejection fraction; NYHA, New York Heart Association; VT, ventricular tachycardia. (Used with permission from the American College of Cardiology. http://www.acc.org/latest-in-cardiology/articles/2015/04/08/09/45/radionuclide-molecular-imaging-in-heart-failure.)

The utility of performing both early and late planar images has been questioned given that the latter provides stronger prognostic utility,40 with an ongoing multicenter prospective study62 requiring only the late images. To improve image efficiency, use of a single 2-hour postinjection planar image has been investigated,63 but the clinical utility of this approach has not been established.
At the same time, a frequently measured planar image parameter that requires both an early and late planar image is 123I-mIBG washout64 which relates to integrity and function of the neuron, but also reflects competition from circulating NE.65 As with HMR, various methods for determining washout have been explored,60 although guideline recommendations exist.30 A normal washout value in control subjects is reported as 10 ± 9%.66 While higher values are associated with worsened prognosis,64,67 the washout variable often drops out in multivariate analyses.40
With regard to SPECT, although most studies examine images processed with filtered back projection, phantom studies suggest that higher image quality is obtained using ordered subsets expectation maximization (OSEM) iterative reconstruction along with deconvolution of septal penetration (DSP) to correct for image contamination from high-energy 123I photons.51 Tomographic 123I-mIBG slices are customarily displayed in a manner similar to that done for MPI,68 and can be shown alongside 99mTc-sestamibi or 99mTc-tetrofosmin perfusion image slices acquired at a separate time in an attempt to detect regions of innervation/perfusion mismatch that may indicate predisposition to ventricular arrhythmias.44,69 Visual image interpretation can be done using scoring methods similar to perfusion SPECT, but quantitation has unique considerations in that while for perfusion images there are usually regions with normal perfusion that can serve as a reference to which count profiles are normalized, with 123I-mIBG, globally reduced tracer uptake is often present. One report used a “mixed reference” method in which classifying a region as “abnormal” varied in relation to global tracer uptake measured by the HMR.70 Another challenge is that unlike for MPI in which validity and accuracy of a methodology is customarily assessed in reference to “gold standard” coronary anatomy findings, there is no such standard upon which to validate adrenergic image-quantitative findings.71 In addition, lung and liver tracer uptake overlying myocardial walls can make SPECT images difficult to interpret fully. Finally, one must also consider that adrenergic imaging assesses a physiologic phenomenon different from perfusion, and thus while more extensive and severe defect(s) on MPI predict poorer outcome, this may not hold for 123I-mIBG imaging in which a more intermediate degree of abnormality that could reflect more electrical heterogeneity may increase predisposition to lethal arrhythmias.72,73
One final issue is that ideally cardiac 123I-mIBG uptake would be homogeneous in normal patients, but some variability occurs in relation to age and gender, as well as from body conditioning, and may be different for SPECT versus PET tracers. Such discussion is beyond the scope of this review, and described in various references.66,74–76 These variations need to be investigated further.
Myocardial innervation imaging has demonstrated the potential benefits in numerous clinical situations, summarized in Table 23-1. While given its widespread prevalence, high mortality, and close association with neurohormonal and sympathetic perturbations, HF with reduced ejection fraction (HFrEF) and associated arrhythmias have been most studied, 123I-mIBG imaging also has demonstrated utility in evaluating patients postcardiac transplant, those with primary arrhythmic diseases, myocardial ischemia and diabetes mellitus (DM), and in monitoring toxic effects of chemotherapy.
|
HF affects more than 5 million Americans and is expected to increase to >8 million by 2030.77 Annual mortality is 40% to 60%, with a cost >$30 billion. While older approaches to HF focused on hemodynamic pathophysiology, the contemporary emphasis is on the neurohormonal model.78 As a result of decreased cardiac function, the sympathetic adrenergic system (SAS) and the renin–angiotensin aldosterone system (RAAS) are activated, that is, at-first beneficially compensatory. However, the processes often become excessive and maladaptive, with deleterious downregulation of β receptors, decreasing myocardial catecholamine levels, and increased plasma NE, with development of anatomic structural changes such as hypertrophy, remodeling, and apoptosis. Continuation of these processes, along with further insults and injury, increase susceptibility to lethal arrhythmias and can lead to a downward clinical spiral toward end-stage HF and death.79
At the sympathetic cellular level, there is an initial increase of NE release into the synaptic cleft, promoting an increase in the NET1 process. Eventually, the system is overwhelmed, with a reduction in carrier density leading to increased spillover of NE into plasma that may account for the increased washout seen with 123I-mIBG imaging in HF patients. As disease becomes advanced, there is diminished presynaptic function from loss of neurons and downregulation of NET1, with decreased global radiotracer uptake and thus a lower HMR.65 HF pathophysiologic processes, as well as insults leading to it such as ischemia and infarction, can cause temporary sympathetic dysfunction that has been referred to as “dysinnervation” (or sympathetic nerve “dysfunction”) that has recovery potential, or “denervation” in which there is anatomic loss of the nerve fibers.80
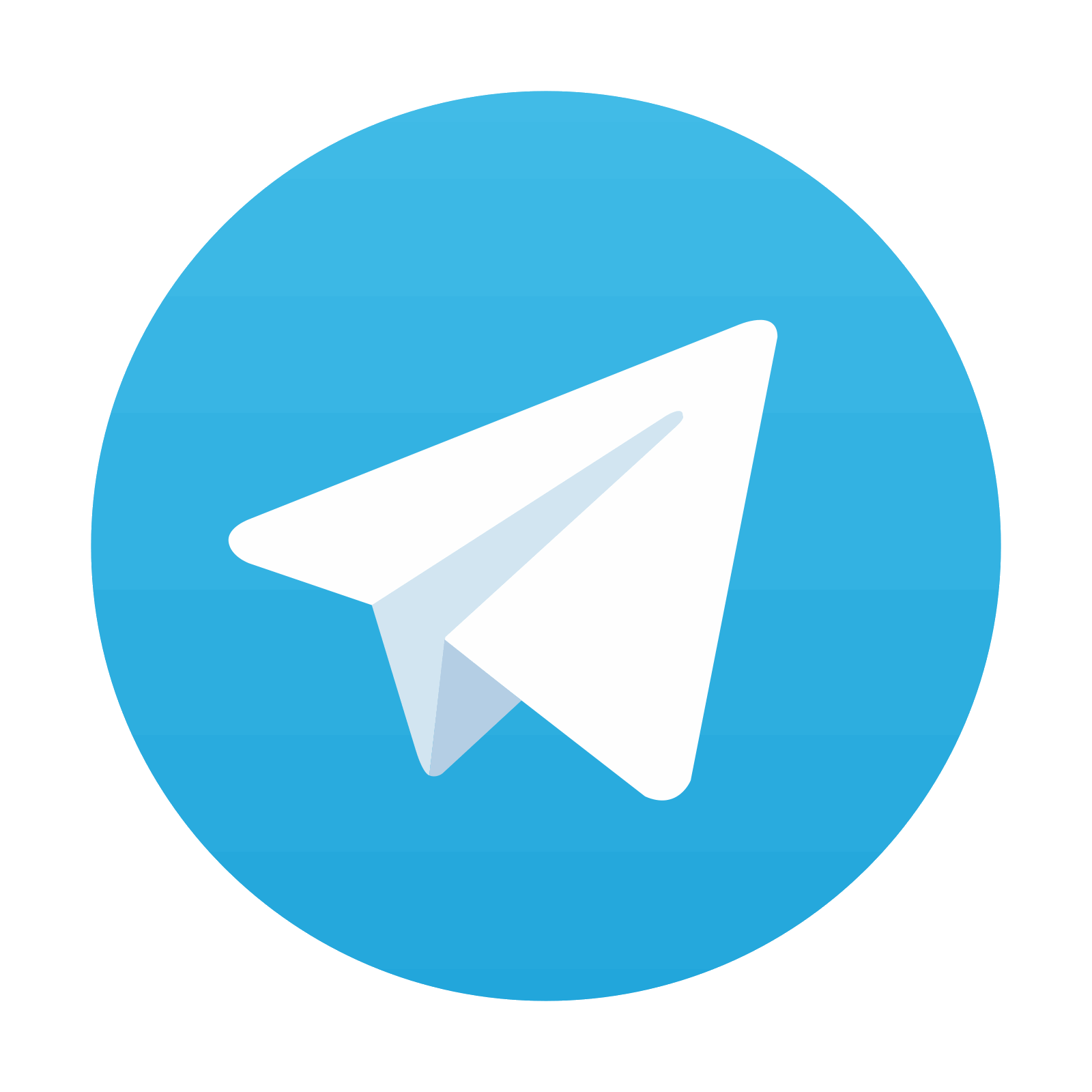
Stay updated, free articles. Join our Telegram channel
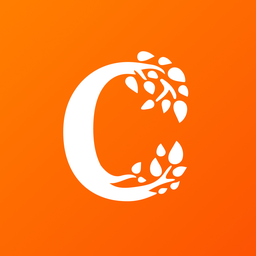
Full access? Get Clinical Tree
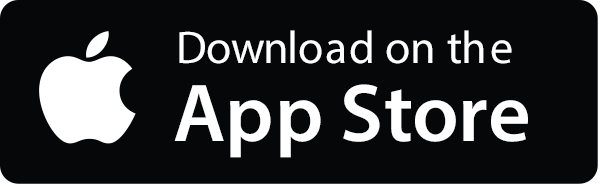
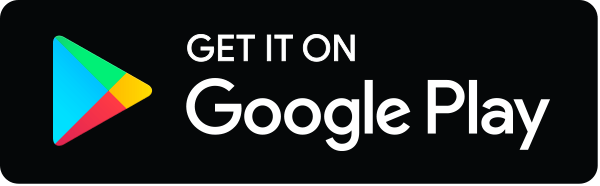