The noninvasive assessment of resting left ventricular (LV) performance is an integral part of the evaluation of patients with known or suspected cardiac disease, having important diagnostic, therapeutic, and prognostic significance.1–11 Although scintigraphic measures of cardiac function historically included measurement of ejection fraction (EF), estimation of cardiac output, valvular regurgitant fraction, and detection of intracardiac shunts have also been successfully performed. Other than EF, these potential applications for nuclear cardiology techniques have been largely supplanted by echocardiography, cardiac computed tomography, and magnetic resonance imaging (MRI) techniques over the past two decades.
Gated equilibrium radionuclide angiography (ERNA, often called multiple-gated acquisition [MUGA] and equilibrium radionuclide ventriculography [RNV]) were introduced nearly 30 years ago. These techniques were routinely utilized in the evaluation of patients with known or suspected LV dysfunction, postmyocardial infarction (MI), and valvular disease, and for monitoring the cardiotoxic effects of chemotherapeutic drugs. Exercise radionuclide angiography (RNA), particularly with the first-pass radionuclide angiographic (FPRNA) technique, was widely used to diagnose or evaluate known coronary artery disease (CAD),5–9 competing favorably with and often complementing planar myocardial perfusion imaging (MPI). However, since its introduction, RNA has evolved little, while other noninvasive methods, such as gated single-photon emission computed tomography (SPECT) MPI, echocardiography, and cardiac magnetic resonance, have been introduced and become increasingly sophisticated and cost effective.
For FPRNA alone, Tc-99m DTPA is often used if no equilibrium images are required. For ERNA, red blood cell labeling can be performed via multiple methods. The in vitro method, usually using a commercial kit, is preferred, and offers the highest level of labeling efficiency (>97%). Blood is withdrawn from the patient, mixed into a “reaction vial” containing stannous pyrophosphate. After 5 minutes it is mixed with sodium hypochlorite to destroy extracellular stannous ion, and then with citrate buffer. After shaking lightly, 25mCi Tc-99m is added, incubated for 20 minutes and then reinjected back into the patient. The in vivo method, although simple, cheap and fast, is no longer recommended as it provides the lowest labeling efficiency (80–85%). It involves giving stannous pyrophosphate directly to the patient, followed by Tc-99m 15 to 20 minutes later (which can be given as a rapid bolus if for FPRNA). Finally, a modified in vivo/in vitro method can be used with labeling efficiency of 92% to 95%. Perfusion imaging can also be performed if desired.12–14
FPRNA images are usually obtained using a single- or multicrystal high-count rate gamma camera fitted with a high-sensitivity parallel hole collimator (e.g., SIM 400, Scinticor, Milwaukee, WI; or ElGems CardiaL [formerly Elscint], Haifa, Israel). Images are acquired in the anterior or the right anterior oblique (RAO) projection using 25 (±4) frames per cardiac cycle. Preliminary work suggests that FPRNA can also be performed with positron emission tomography (PET) using images acquired during a bolus of radiolabeled water (H215O), but this has not yet achieved widespread utilization or validation.15
FPRNA data are analyzed using the frame method for LVEF using commercially available computer software,16–18 as shown in Figures 22-1, 22-2, 22-3. This software creates a representative LV volume curve by summing frames of several (usually 5–10) cardiac cycles, which are aligned by matching their end diastoles (histogram peaks) and end systoles (histogram valleys) during the operator-defined levophase of radioactive tracer transit. The pulmonary-frame background-corrected representative cycle is then examined with a fixed region of interest (ROI) in order to obtain the final first-pass LV time–activity curve. This ROI is drawn over the LV as defined by a first harmonic Fourier transformation phase image, which distinguishes clearly the LV from the aortic counts. End diastole is taken as the first frame of the representative cycle, and end systole is defined as the frame with the minimum counts in the histogram. Historically, the LVEF was taken as the end-diastolic counts minus the end-systolic counts, divided by the background-subtracted end-diastolic counts.
Figure 22-1
FPRNA (anterior projection) images are shown, with the serial images at the lower left, demonstrating tracer transit from the superior vena cava, to the right atrium, to the right ventricle to the pulmonary phase, left heart phase, and systemic circulation. Using regions of interest (ROIs) drawn over the LV and left lung (far upper left image), histograms are obtained (shown above serial images), which show overlapping RV counts with systoles (curve valleys) and diastoles (curve peaks) from which the cardiac cycles (CYC) are derived that comprise the representative cycle. Each cardiac cycle is marked. The pulmonary curve is used to compute the pulmonary mean transit time (PMTT). The length of the representative cycle in frames (FR) is used to derive the heart rate (HR). The images of the raw representative cycle are shown at upper right. This is subjected to the frame method of background subtraction (i.e., using the background to end-diastolic image ratio [BG/ED] and the washout factor [WO] needed to set the pulmonary area to zero counts), in order to derive the corrected representative cycle (upper left images) from which single ROI ejection fraction (SNGL EF), which is higher than the raw EF, but lower than the dual ROI-derived EF, which is used to account for valve plane motion. The Fourier amplitude (AMP) and phase (PHS) images at the lower right demonstrate reduced apical amplitude and delayed contraction of the apex, respectively.

Figure 22-2
FPRNA (anterior projection) functional images are shown, with end-diastolic and end-systolic perimeter images at the upper left (ED – ES), a paradox image (lower left), regional ejection fraction index (REFI, upper right), and Fourier phase images (lower right) shown. The Fourier phase image demonstrates delayed contraction of the majority of the apex, with a small area of paradoxical movement (aneurysmal) evident at the apex on the paradox (ES counts – ED counts) image. These functional images allow assessment of regional function without the need for visual interpretation of cine images.

Figure 22-3
Additional FPRNA (anterior projection) functional images are shown, with regional ejection fraction index for the first and second halves of systole (REFI 1 and REFI 2, upper frames), an alternative method of determining the presence of delayed contraction. Note that the apical region has more ejection fraction in the latter half of systole, compared with the inferobasal wall. Fourier amplitude (AMP, lower left) and stroke volume (SV, ED – ES, lower right) images are shown. The graphic extending from the valve plane to the apex on the SV image is used to compute the LV volume using the Sandler and Dodge equation for the anterior projection.

A second ROI (end diastolic) can be derived from a Fourier transformation amplitude image with masking of the lower 10% of image intensity, which extends the ROI in a basal direction, usually one to four pixels, depending on the vigor of ventricular contraction, up to the amplitude signal of the aortic root. The remainder of this ROI is drawn to match the first ROI (end systolic). The dual ROI LVEF is determined as the end-diastolic ROI counts minus the end-systolic ROI counts, divided by the background-subtracted end-diastolic ROI counts. This results in an accounting for valve plane motion during the cardiac cycle, using Fourier-guided dual ROI analysis of FPRNA, giving EFs that are highly reproducible and similar in value to ERNA.18
Electrocardiogram (ECG)-gated planar equilibrium blood pool images (Fig. 22-4) can be performed with any of the several blood pool agents, such as Tc-99m–labeled red blood cells (described above and the standard) and Tc-99m–labeled human serum albumin. These images are best when acquired using high-resolution collimation. Images for planar LVEF calculation are obtained in the best septal (shallow) left anterior oblique (LAO) view. For regional wall motion assessment, the best septal view ±45 degrees should be obtained (approximately “anterior” and “lateral” views). Each of these planar-gated images should be acquired for 6- to 10-minute duration, dividing each cardiac cycle into a minimum of 32 frames. A smaller number of frames (e.g., 16) are adequate for assessment of EF due to the usual length of the isovolumic ejection period (at end diastole) and relaxation period (at end systole). However, more frames are desirable if quantitative assessment of diastolic performance is needed.
Figure 22-4
ERNA analysis is shown for images obtained in the LAO 45-degree projection. The 32 ECG-gated frames are analyzed using a guiding ROI (frame 1) obtained either manually or using Fourier phase and amplitude images to automatically locate and outline the LV. Automated LV edge detection is performed using a combination of first and second derivatives of count profiles inside the guiding or master ROI. Background correction is performed based on the counts per pixel within a small periventricular ROI (frame 16) drawn carefully to avoid the ventricle or the spleen. The counts within the 32 ROIs are shown after background correction in the lower left histogram. The first derivative of this ventricular volume curve is used to compute the peak filling and emptying rates (PFR and PER). The Fourier phase and amplitude functional images demonstrate inferoapical and septal hypokinesis with late contraction, when compared with the RV and the basal lateral portion of the LV.

For LVEF, automated regions of interest are generated on the planar-gated equilibrium blood pool data throughout the cardiac cycle using the commercially available software. An automated periventricular background ROI must be adjusted routinely in order to avoid inclusion of high-count structures (e.g., spleen and descending aorta), which would artifactually increase the LVEF if included.
ECG-gated projections for SPECT reconstruction are usually obtained using high-resolution collimation in a fashion similar to gated SPECT perfusion imaging (Fig. 22-5), scanning from RAO 45 degrees to left posterior oblique (LPO) 45 degrees projections. A total of 45 to 60 projections of 20- to 30-second duration, at 4-degree or 3-degree steps, respectively, should give adequate count density. At each projection, either 8 or 16 frames per cardiac cycle should be acquired. After collimator sensitivity and center of rotation correction, projections are reconstructed into transaxial slices of the blood pool for each of the 8 or 16 frames of the cardiac cycle. The transaxial slice sets can then be reoriented in cardiac planes (i.e., short axis, horizontal long axis, and vertical long axis) for each of the eight frames of the cardiac cycle.
Figure 22-5
Gated tomographic ERNA analysis is shown after three-dimensional reconstruction of the left and right ventricles and surface rendering. This display (BPQS, Cedars-Sinai, Los Angeles) is termed Splash3D. All three synchronized pairs of 3D views are displayed. The lower views can be gated, and each of these views can be rotated interactively. It is also possible to superimpose an isosurface in this mode for rapid display of regional wall motion.

Multiple methods of quantifying blood pool SPECT images have been published using either three-dimensional images or midventricular horizontal and vertical long-axis slices, which can be analyzed for wall motion or EF.19–25 The LVEF and RVEF can be calculated for each long axis as the end-diastolic counts minus the end-systolic counts, divided by the end-diastolic counts, combining them for a biplane EF.
The three-dimensional techniques are useful for blood pool volume rendering, which is used for delineation of myocardial (actually endocardial) topography. This technique is limited by the accuracy of automated valve plane definition since there is often little count density change at the mitral or tricuspid valve planes. However, this method has been validated against standard approaches, such as cardiac magnetic resonance.25 Due to the uniformly high contrast and lack of overlap between cardiac blood pool and extracardiac structures, no background subtraction algorithm should be employed for these analyses.
Gated SPECT MPI (Fig. 22-6) has become one of the most powerful tools available in nuclear cardiology and is discussed in detail in Chapter 11. Acquisition of SPECT data, synchronized with the electrocardiographic R wave, is generally performed using 8 or 16 gating intervals and allows evaluation of both global (EF) and regional (myocardial wall motion and wall thickening) cardiac function. Gated SPECT acquisition is now recommended for all perfusion studies whenever possible. It is estimated that over 90% of all SPECT studies in the United States are currently performed using the gated acquisition technique.26 Gated SPECT MPI is most often performed with poststress ECG gating. Ungated SPECT perfusion imaging is usually reserved for resting images in some institutions or for patients with cardiac arrhythmias.
Figure 22-6
Gated SPECT myocardial perfusion images are shown analyzed with the commercially available QGS program (Cedars-Sinai) used for display and automated calculation of ejection fraction and volume. Changes in volume are tracked from ED to ES, for calculation of wall motion and regional thickening, displayed in polar map format. Three-dimensional surface-rendered diagrams (above) and actual SPECT slices with fitted edges (below) are also shown. These images were obtained with Tl-201 in a normal patient.

Two- and three-dimensional techniques for display of gated perfusion SPECT have been well validated for EF.26–31 Gated SPECT has been utilized extensively for determination of EF and wall motion, adding incremental diagnostic and prognostic information. The addition of wall motion has improved the specificity of myocardial perfusion SPECT by distinguishing myocardial scarring from attenuation artifacts, both of which may result in “fixed” defects.32
The exponential increase in the use of gated SPECT perfusion has been fueled by the increased availability of automatic and semiautomatic algorithms for the quantification of cardiac function. DePuey et al.29 described a method based on the automated detection of endocardial borders. Williams and Taillon28 developed a method based on digitally subtracting and inverting the perfusion images and analyzing the change in counts occurring in the ventricular chamber, using an edge-detection method identical to that commonly used in gated ERNA. Germano et al.27,30 developed a totally automated method of fitting geometric shapes to the endocardial borders to obtain systolic and diastolic volumes and EFs. Smith et al.31 have used partial volume effect to quantitate regional thickening and estimate LVEF, without need for any edge-detection algorithm. Each of these techniques has been well “correlated” with standard methods of performing EF, but each demonstrating varying degrees of “substitutability.”28
For these reasons, the gated SPECT policy statement of the American Society of Nuclear Cardiology encourages the use of gated SPECT with every perfusion study in which gating is feasible. This reflects the improved quality control and diagnostic accuracy of interpreting perfusion images in light of regional and global LV performance, as well as the incremental value of gated SPECT over perfusion imaging alone for prognosis.32–35
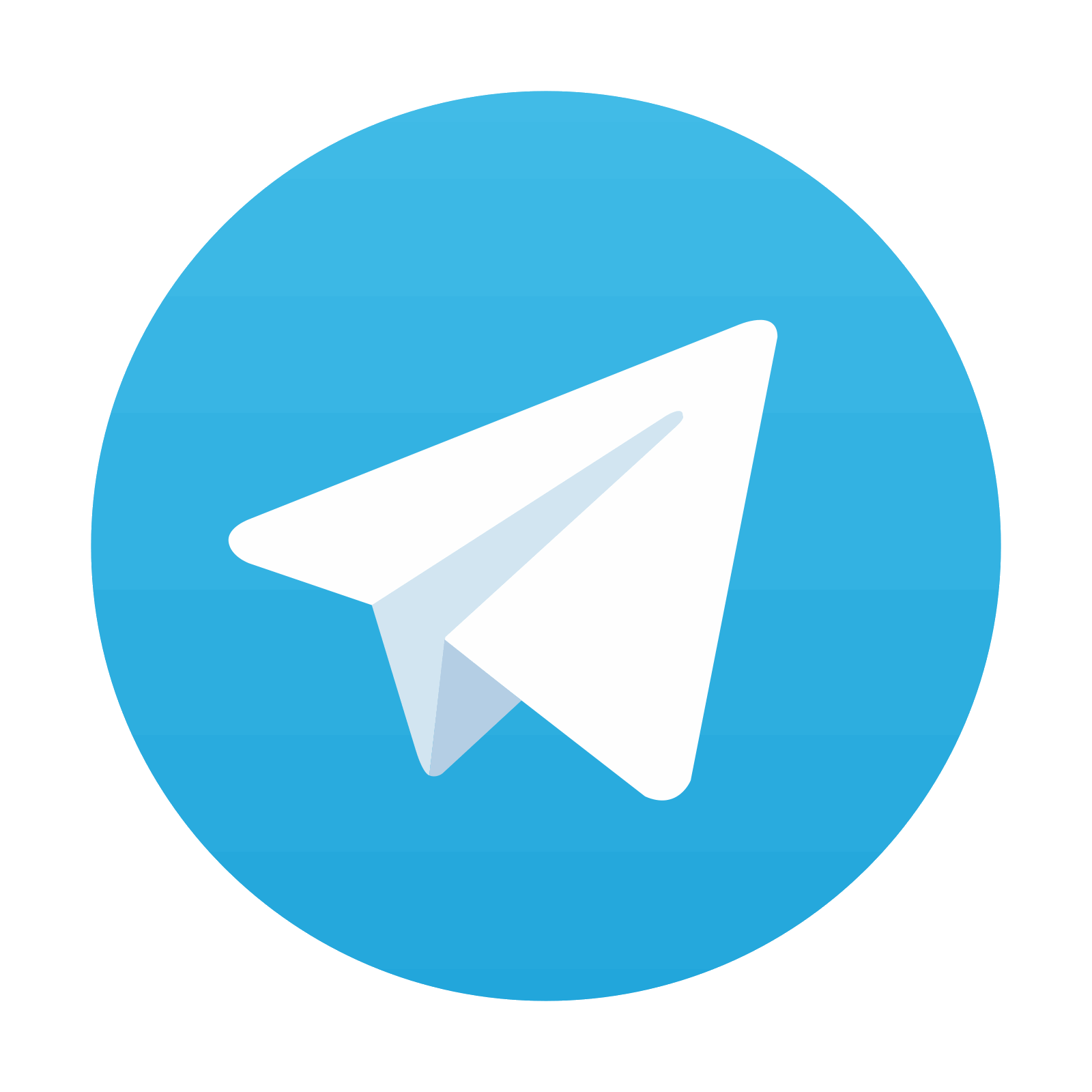
Stay updated, free articles. Join our Telegram channel
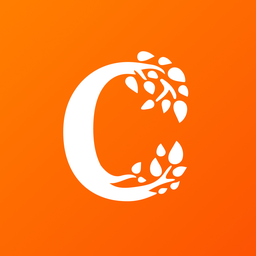
Full access? Get Clinical Tree
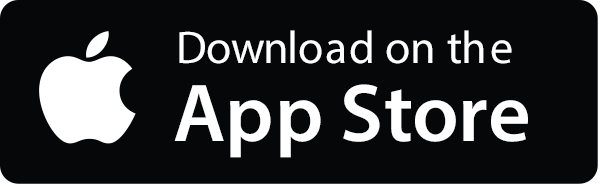
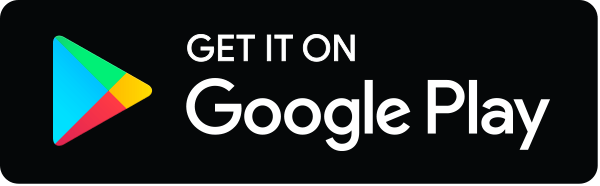