The use of radionuclides for the assessment of cardiovascular disease is well-established as a very low-risk, high-sensitivity, and specificity technology for establishing the presence of coronary disease, hibernating myocardium, and other cardiac and cardiovascular syndromes.1,2 Recently societies and professional medical organizations have expressed concern for radiation exposure to patients undergoing nuclear cardiology procedures.3 It is important in addressing concerns of radiation exposure that the quality of nuclear cardiology procedures not be sacrificed for the purposes of reducing the patient’s radiation dose alone. Nuclear cardiology procedures can confer life-saving benefits; however, the application of sensible radiation reduction techniques can maintain and even improve the diagnostic quality of nuclear cardiology images while reducing the patient dose.3–6
Current understanding of the influence of radiation on the patient’s long-term risk assumes the effect of ionizing radiation is cumulative over a patient’s lifetime. More recent genomic studies of double strand breakage and repair mechanisms may indicate a simple linear; no threshold model for the influence of radiation may overestimate the risk of carcinogenesis from diagnostic radiation.7 Despite this, each procedure must take into account radiation-reducing steps to have an impact on the overall patient dose. However, radiation-reduction strategies should not come at the expense of diagnostic quality.
Imaging societies encourage nuclear laboratories and health-care providers to consider patient needs when prescribing imaging tests.8 For example, elderly patients with known coronary disease have a different risk-to-benefit relationship than younger patients at a lower likelihood for coronary disease. Customizations of imaging protocols should take into account patients’ needs for an accurate assessment of cardiovascular disease in addition to their own sensitivity to ionizing radiation, as well as the frequency of testing procedures being ordered.
Cardiac PET offers some unique advantages over SPECT by simultaneously providing improved image quality, diagnostic accuracy, and reducing patient dosage.9 This chapter investigates the trade-offs of reducing patient dose, specific advantages of cardiac PET, and recommends strategies for optimizing patient dose and image quality with a cardiac PET procedure.
Photon ionizing radiation interacts with cells by depositing energy in a focal region, disrupting the cell’s machinery. Of greatest concern is changes in the cell’s DNA that could lead to carcinogenesis. At lower photon energies (ultraviolet radiation), radiation can cause focal breakages of DNA base pairs, resulting in pyrimidine dimers that then can be sites of incorrect or incomplete DNA repair.10 These incomplete repairs have the potential for producing viable cancerous cells as is the case of melanoma.
At higher photon energies, the mechanism for carcinogenesis is the production of hydroxyl radicals.11 When gamma radiation interacts with molecules inside of a cell, the result is a Compton scattered electron that thermalizes in the medium. This thermalization process produces free electrons that break the hydrogen-hydroxide bond leading to free hydroxyl radicals inside of the cell. These hydroxyl radicals interact with the DNA, typically by breaking either a single or double strand of the DNA. In most cases single or double strand breakages of the DNA do not result in a viable cell, or the DNA strand is repaired.
The most common model for estimating radiations role in carcinogenesis is the “linear no threshold model.”12,13 This model assumes that radiation risk and the potential for carcinogenesis can be linearly extrapolated from high-dose examples of carcinogenesis. These high-dose examples, such as the atomic bomb blast victims in Hiroshima and Nagasaki, the Chernobyl nuclear accident, Fukushima nuclear accident, and so on, represent the limited experience of direct causal relationship between ionizing radiation and carcinogenesis. The linear no threshold model assumes that the incidence of radiation-induced cancer at these high-dose exposures can be extrapolated to the very low doses observed in diagnostic radiological exams. This is generally accepted as an upper bound of the risks of radiation exposure. These high-dose models differ significantly from diagnostic radiological exams in a number of ways. First, the source of radiation in most of these models is neutron radiation not photon radiation. Neutron radiation has been shown to have significantly higher capacity for cellular damage because of the intense deposition of ionization in a very small region (high-linear energy transfer, LET). In addition, the high-dose radiation overwhelms the cells natural systems for repairing damaged DNA.
Recent investigation of carcinogenesis as a result CT radiation showed DNA damage at varying levels of CT exposure.14 This study investigated particular biomarkers for DNA damage: phosphorylated H2AX, ataxia telangiectasia mutated (ATM), and tumor protein p53. The study also investigated markers of apoptosis and BCL2 associated X protein (BAX). This study concluded that radiation exposure typically results in cell death and activation of DNA repair processes. Although it did not evaluate carcinogenesis specifically, the authors were able to conclude that higher energy ionizing radiation most commonly results in noncancerous by-products. In addition to limited evidence of double strand breaks in Nguyen 2015, mutational signatures for both leukemia and lymphoma do not exhibit the broad mutational spectrum associated with double strand breakage (Figure 8-1). Additional whole genome data is needed to establish an upper bound on the prevalence of double strand breakage and repair in patients with leukemia and lymphoma.
Figure 8-1
The Drivers Of Carcinogenesis Are Somatic Mutations To The DNA Of Patients Environmental Exposures leave specific signature on the characteristic mutations on DNA. For leukemia, there are only two particular mutations that are known to create these carcinomas and they do resemble the typical genetic markers associated with double strand breakage and repair.

These and other data suggest that nuclear laboratories should continue to strive to make reductions in patient radiation exposure whenever possible. However, because of uncertainty in the relationship between radiation, carcinogenesis and optimal patient care, efforts to reduce patient radiation exposure should not come at the expense or sacrifice of the diagnostic utility of an imaging test.
In order to describe ionizing radiation, it is necessary to first establish the units of measurement. For radioactivity these measurements are usually made in terms of Curie or Becquerel. Both Curie and Becquerel are measurements of the number of disintegrations of radioactive atoms per second, or activity. One Becquerel of radioactive material is defined as one atomic disintegration per second. The Curie is also based on the number of disintegrations per second (3.7 × 1010 disintegrations/sec). In nuclear cardiology, measurements are typically made in terms of millicurie (mCi) or megabequerel (MBq). One mCi is equal to 37 MBq.
Radiation dose is described in one of two ways: amount of energy deposited per cubic centimeter of tissue and amount of tissue damage per cubic centimeter of tissue. The measurement of the energy deposited per cubic centimeter is the Gy defined, which is 1 J per kilogram absorbed ionizing radiation. More common in diagnostic imaging is the rad, which is 1/100 of a Gy. Equivalent dose (H) takes into consideration the relative biological damage of ionizing radiation. It is the product of the absorbed dose (D), times a weighting factor for the source of radiation (1 for gamma rays), times a weighting factor for the sensitivity of the particular organ to ionizing radiation. The units of equivalent dose are Sieverts (Sv) or roentgen equivalent man (rem). For whole body, this can be summarized as the effective dose (E), which is a weighted sum of all of the individual dosages to the organs (ICRP). The adult organ and total body dosimetry values are usually based on an idealized 70 kg person.15 To compare radiation exposure between modalities or even radiotracers, most professional societies have adopted the mSv as a means of communication potential risk to patients.
The most important current cardiac PET tracers are rubidium 82, 13N-ammonia, and F-18 labeled FDG. In addition to these, several other important radionuclides are under research investigation: F-18 flurpiridaz and O-15 water. All of these tracers have the radiological advantage of a very short half-life. This short half-life is one of the key advantages PET tracers have over SPECT tracers in reducing patient radiation exposure. SPECT tracers, such as sestamibi and tetrofosmin, have biological half-lives (6 hours) much greater than the acquisition time, causing patients to continue receiving radiation exposure long after the conclusion of the diagnostic testing. In contrast, PET tracers typically decay to background soon after the conclusion of testing thus minimizing unused radioactive decays. A second advantage of cardiac PET tracers is 511 keV photon pair produced during positron annihilation travel in opposite directions, eliminating the need for collimation. This advantage provides much more efficient data collection thereby reducing the need for high injected activity. A summary of the radiation dose from PET tracers is given in Table 8-1.
Study Protocol | Isotope | Modality | Activity (mCi)† | E (mSv)†† |
---|---|---|---|---|
Rest + Stress Perfusion | 82Rb-chloride | 3D PET | 25 + 25 | 2 |
2D PET | 50 + 50 | 4 | ||
Rest + Stress Perfusion | 13N-ammonia | 3D PET | 10 + 10 | 2 |
2D PET | 20 + 20 | 4 | ||
Rest Viability, Sarcoid or Inflammation (+ Perfusion) | 18F-FDG (+13NH3 or 82Rb) | 3D PET | 5 (+10 or 25) | 3.5 (+ 1) |
2D PET | 10 (+20 or 50) | 7 (+ 2) | ||
Stress-only Perfusion (ultra-low-dose) | 99mTc-sestamibi | CZT-SPECT | 3.5 | 1 |
Stress-only Perfusion (full-dose) | 99mTc-sestamibi | GC-SPECT | 30 | 10 |
Rest + Stress Perfusion One-day (half-dose) | 99mTc-sestamibi 99mTc-tetrofosmin | GC-SPECT | 5 + 15 | 6.4 5.6 |
Rest + Stress Perfusion One-day (full-dose) | 99mTc-sestamibi 99mTc-tetrofosmin | GC-SPECT | 10 + 30 | 13 11 |
82Rb is a 76-second half-life potassium that is produced by a 82Sr-82Rb generator that is eluted by an infusion pump system into the patient.16,17 The eluate from the 82Sr-82Rb generator must be checked daily to ensure that any contamination with long-lived 82Sr and 85Sr is below acceptable limits.
Radiation dosimetry measurements of 82Rb have been difficult because of its very short half-life. Current estimates acquired on modern PET/CT imaging equipment and using improved kinetic modeling of 82Rb now estimate the radiation dose from a typical rest stress perfusion 82Rb study has been less than one-fourth the dose from Tc 99m myocardial perfusion SPECT studies (12–15 mSv for SPECT, 3–5 mSv for 82Rb PET18–20).
A significant contributor to the radiation dose from 82Rb is the energetic positron that is emitted during decay (max energy 3.3 MeV). This positron’s energy is deposited through thermalization in the tissue. This high-linear energy transfer of the positrons’ kinetic energy increases the possibility of cellular damage. In addition to the high-kinetic energy of the admitted positron, 12% of 82Rb decays also admit a secondary “prompt gamma” photon at 776 MeV. These additional contributors to ionizing radiation increase 82Rb’s potential for causing cellular damage.
13N-ammonia is also commonly used for myocardial perfusion PET studies, and has several advantages of 82Rb. 13N-ammonia is a highly extracted tracer with a 9.93 minute half-life.21 This longer half-life can enable both pharmacological and exercise testing, and can be delivered short distances as a unit dose.
The amount of ionizing radiation deposited in tissue per disintegration is less with 13N-ammonia versus 82Rb. The maximum kinetic energy of the emitted positron is 1.2 MeV with no prompt gamma. However, given the longer half-life of 133ammonia the radiation dose from 13N-ammonia is comparable to clinical doses of 82Rb (Table 8-1).
18F 2-fluorodeoxyglucose (18F FDG) is also commonly used in cardiac PET procedures, but is not a blood flow tracer. Its most common application is for the detection of myocardial viability. In addition to viability studies, 18F FDG is also used for the detection of sarcoidosis and inflammation. 18F is usually produced in a cyclotron by proton irradiation of 18O water and has a 109.8 minute half-life. The deoxyglucose is then labeled with 18F to yield 18F FDG, an analog of glucose.22 Similar to 13N-ammonia, FDG produces a low-energy positron (0.633 MeV), with no prompt gamma by-product.
Several PET perfusion tracers labeled with 18F have been under active development for the past few years: a fluorinated perfusion agent, Flurpiridaz.23,24
The injected activity from 18F-flurpiridaz tracer in phase II and phase III clinical trials has been approximately 1.9 mCi at rest and 6.5 mCi at stress for the exercise study and 2.9 mCi for rest and 6.8 mCi for the pharmacologic study (Figure 8-2). Phase 1 studies of Flurpiridaz23 estimate the radiation dose as approximately 6.4 mSv and 4.8 mSv for the pharmacologic and exercise rest/stress protocols, respectively. Because of the longer half-life of F-18, the radiation dose from flurpiridaz is higher than 82Rb or 13N-ammonia, although some studies have indicated that flurpiridaz could be effectively imaged using a lower injected dose. Even these levels of the radiation exposure is well below ASNC recommendations. In addition, exercise stress-only protocols will also be investigated to reduce dose. Either by using a low-dose stress high-dose rest study or by using a 2-day study, it may be possible to achieve a less than 2 mSv protocol in normal patients.
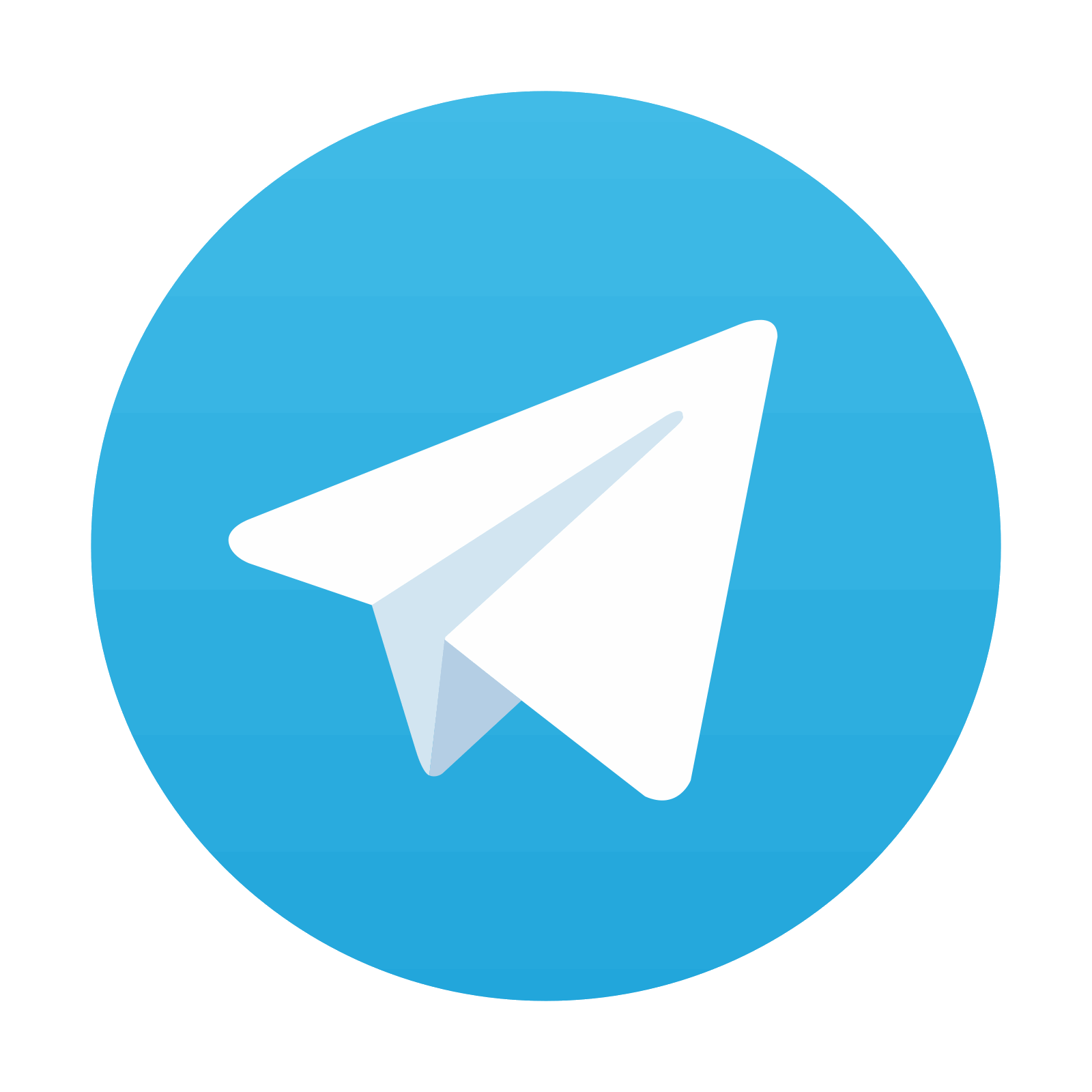
Stay updated, free articles. Join our Telegram channel
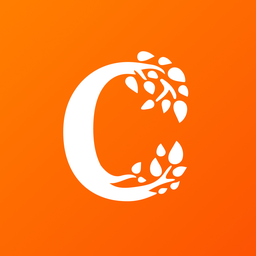
Full access? Get Clinical Tree
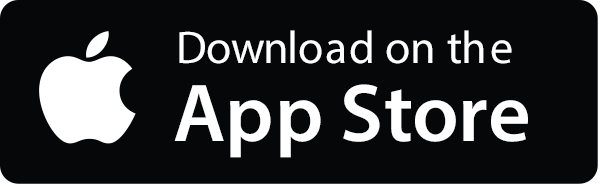
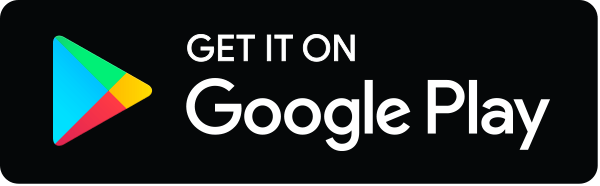