Educational Aims
The reader will come to appreciate that:
- •
Race-based disparities in pulse oximetry have been reported in large retrospective database studies involving adults and recently in pediatric populations.
- •
Pulse oximeters overestimate oxygen saturation in Black compared to other races, particularly in hypoxic states.
- •
Role of skin pigmentation and other dermatological factors that may vary between racial groups has not been evaluated.
- •
Defining mechanisms of this inaccuracy is critical to addressing this disparity in pulse oximeter performance.
Abstract
Race-based and skin pigmentation-related inaccuracies in pulse oximetry have recently been highlighted in several large electronic health record-based retrospective cohort studies across diverse patient populations and healthcare settings. Overestimation of oxygen saturation by pulse oximeters, particularly in hypoxic states, is disparately higher in Black compared to other racial groups. Compared to adult literature, pediatric studies are relatively few and mostly reliant on birth certificates or maternal race-based classification of comparison groups. Neonates, infants, and young children are particularly susceptible to the adverse life-long consequences of hypoxia and hyperoxia. Successful neonatal resuscitation, precise monitoring of preterm and term neonates with predominantly lung pathology, screening for congenital heart defects, and critical decisions on home oxygen, ventilator support and medication therapies, are only a few examples of situations that are highly reliant on the accuracy of pulse oximetry. Undetected hypoxia, especially if systematically different in certain racial groups may delay appropriate therapies and may further perpetuate health care disparities. The role of biological factors that may differ between racial groups, particularly skin pigmentation that may contribute to biased pulse oximeter readings needs further evaluation. Developmental and maturational changes in skin physiology and pigmentation, and its interaction with the operating principles of pulse oximetry need further study. Importantly, clinicians should recognize the limitations of pulse oximetry and use additional objective measures of oxygenation (like co-oximetry measured arterial oxygen saturation) where hypoxia is a concern.
Introduction
Pulse oximeters are essential tools for vital sign monitoring and oxygen saturation assessment. Skin color-based pulse oximeter inaccuracy, especially at low oxygen saturation, has been known for over a decade . The COVID-19 pandemic and integration of large multi-institutional electronic health system data sets have heightened the awareness of a racial disparity in pulse oximeter inaccuracy among adults and raised concerns about its impact on amplifying health and healthcare-related inequities and disparate clinical outcomes . More recently, studies in infants and children across diverse settings and age ranges have also highlighted systematic inaccuracies in the accuracy of pulse oximetry based on racial groups. Infants and children constitute a particularly vulnerable population at an inherently higher risk of detrimental effects of hypoxia or hyperoxia . Thus, pulse oximeter inaccuracy in these populations could have profound effects on patient outcomes.
The growing literature in adults suggests not only a numerical inaccuracy in pulse oximeter performance across racial groups but also worse clinical outcomes associated with race-based pulse oximeter disparities. Given the length of time many early preterm neonates require support and hospitalization in the neonatal ICU, the effects of race-based or pigmentation-related biased pulse oximeter measurements may be even more substantial. Infants and children may not communicate the extent of their illness, and reliance on objective data for assessment of respiratory illness and risk stratification is of paramount importance. The duration of reliance on pulse oximetry for continuous vital sign assessment is much longer for infants and children than for adults. When compared to the average adult ICU stay of 3.4 days , a length of stay of 5 days for pediatric ICU patients , 2 weeks for term neonates and several months for premature infants, highlights greater reliance on pulse oximetry in the smallest and most fragile patients. The adverse consequences of hypoxia or hyperoxia during a prolonged hospital stay on the developing lung, brain, and heart cannot be overstated .
In this review, we first outline the working principle of a pulse oximeter. We then describe physico-optical interactions and corresponding clinical states that can affect pulse oximeter accuracy. Next, we summarize the key statistical measures used in reporting pulse oximeter performance, with an emphasis on requirements mandated by regulatory organizations. We synthesize the recent literature on racial bias in pulse oximetry in infants and children, followed by potential implications in special circumstances that are unique to pediatric patients. Finally, we present challenges and opportunities to systematically and rigorously quantify this bias in ways that transcend race-based classification.
Principle of operation of pulse oximeters
Heart rate monitoring by pulse oximetry is based on the principle of photoplethysmography whereby light-absorption increases due to the systolic increase in arterial blood volume at the site of device placement (pulsatile or “Alternating Current” AC compartment) ( Fig. 1 a, b) . The absorption of light by human tissues including skin, subcutaneous fat, muscles, bones, and capillary and venous blood is stable over the timeframe of a pulse oximeter measurement (non-pulsatile or “Direct Current” DC compartment) . Therefore, any changes in light absorption at the site of oxygen measurement are due to variations in the arterial blood volume related to the cardiac cycle. Determination of arterial hemoglobin oxygen saturation by pulse oximetry (SpO 2 ) is based on the spectral characteristics of oxygenated and deoxygenated hemoglobin with regard to light absorption in the red and infrared (IR) wavelengths . Currently employed pulse oximeters have 2 light-emitting diodes, one emitting at 660 nm (red), where deoxygenated hemoglobin is the dominant absorber, and the other at 940 nm (infra-red), where oxygenated hemoglobin has higher absorption than deoxygenated hemoglobin. The non-absorbed (or transmitted) energy is detected by a sensor with an inbuilt microprocessor. Using Beer-Lambert’s law relating absorption to concentration of absorbers, the microprocessor subtracts the absorption by a constant ( Fig. 1 a, b). The SpO 2 is then calculated from the absorption ratio R which is a double ratio of the pulsatile (AC) and non-pulsatile (DC) components of red light absorption to IR light absorption and is calculated as <SPAN role=presentation tabIndex=0 id=MathJax-Element-1-Frame class=MathJax style="POSITION: relative" data-mathml='Ared ACred DCIRACIRDC’>𝐴red ACred DC𝐼𝑅𝐴𝐶𝐼𝑅𝐷𝐶Ared ACred DCIRACIRDC
A red AC red DC I R A C I R D C
where A = absorbance ( Fig. 1 c). The microprocessor of the device maps this ratio R to SpO 2 values from a calibration algorithm derived from simultaneous SpO 2 and co-oximetry measured SaO 2 in healthy adult volunteers breathing mixtures of decreased oxygen concentrations in controlled lab settings . The exact calibration algorithms are proprietary and specific to each manufacturer.

Blood oxygen saturation (SaO 2 ) – Functional and fractional SaO 2
Measurement of arterial blood oxygen saturation can be represented by two concepts, functional SaO 2 and fractional SaO 2 , which are important to distinguish while discussing pulse oximeter validation . The term “functional SaO 2 ” used in pulse oximeter validation is defined based on concentrations of oxyhemoglobin (O 2 Hb) and deoxyhemoglobin (HHb):
SaO2func=O2HbO2Hb+HHb
SaO 2func is the proportion of blood that is oxygenated (O 2 Hb) in relation to blood that could have been saturated with oxygen (O 2 Hb + HHb). SaO 2func is distinguished from “fractional SaO 2 ” (SaO 2frac ), which refers to the concentration of oxyhemoglobin (O 2 Hb) divided by total hemoglobin, which is the sum of O 2 Hb + HHb + dyshemoglobins that lack the ability to bind oxygen like carboxyhemoglobin (COHb) and methemoglobin (MetHb).
SaO2frac=O2HbO2Hb+HHb+COHb+MetHb
Sa O 2 f r a c
better estimates the oxygen carrying capacity of blood, in the absence of clinically significant amounts of COHb and MetHb, <SPAN role=presentation tabIndex=0 id=MathJax-Element-5-Frame class=MathJax style="POSITION: relative" data-mathml='SaO2func’>Sa𝑂2𝑓𝑢𝑛𝑐SaO2func
Sa O 2 f u n c
is a valid comparison for SpO 2 and is used in device calibration studies since both <SPAN role=presentation tabIndex=0 id=MathJax-Element-6-Frame class=MathJax style="POSITION: relative" data-mathml='SaO2func’>Sa𝑂2𝑓𝑢𝑛𝑐SaO2func
Sa O 2 f u n c
and SpO 2 functionally measure just the oxy- and de-oxyhemoglobins.
Factors affecting pulse oximeter accuracy
The accuracy of a pulse oximeter is evaluated by the differences between SpO 2 (the oxygen saturation values reported by the pulse oximeter) and SaO 2 (measured by co-oximetry in extracted blood that is considered the gold standard) . Awareness of basic principles of calibration and validation of pulse oximeters will provide clinicians with a conceptual basis of appreciating pulse oximeter measurement limitations. Pulse oximeter validation studies were based on SaO 2 measurements in healthy young volunteers over a range of desaturation values (SpO 2 70–100 %). Because it is unethical to desaturate volunteers below SaO 2 levels of 70–80 %, lower SpO 2 values are derived by extrapolation and, therefore, are less accurate . Moreover, because the subjects recruited for calibration purposes were mostly healthy young adults (defined as nonsmoking subjects without lung disease, obesity, or cardiovascular problems), the applicability of calibration data to patients at the age extremes and the critically ill has been challenged . Table 1 summarizes technical and physiological factors that are well-known to affect pulse oximeter accuracy.
Factors | Mechanism | Effect |
---|---|---|
Motion | Increased signal-to-noise ratio due to changes in non-pulsatile component of light absorption | ↓ SpO 2 readings False alarms |
Poor perfusion | Decreased signal caused by decreased pulsatile (arterial) component of light absorption | ↓ SpO 2 readings |
Calibration | Device-specific calibration algorithms derived by correlating light absorption ratios over a SaO 2 range 80–100 % in healthy young adults | SpO 2 readings in hypoxic states (<80 %) are less accurate |
Time lag | Software-related delay between sudden changes in blood oxygenation and SpO 2 readings | Delay in detecting clinically important desaturation, which may exceed 20 s |
Probe positioning | Emitted light projects tangentially to the detector | ↓ SpO 2 readings |
Ambient light interference | Intense external light (e.g., phototherapy) may interfere with the photodetector | ↓ SpO 2 readings |
Dyshemoglobins | COHb absorbs red light (like O2Hb) | ↑ SpO 2 readings in significant carboxyhemoglobinemia |
MetHb absorbs equal amounts of red and infra-red wavelengths; affects ratio of absorption | SpO 2 shifts towards 85 % in significant methemoglobinemia | |
Intravenous dyes | Methylene blue, indo-cyanin green, and indigo carmine interfere with light absorption | ↓ SpO 2 readings |
Jaundice | Bilirubin presents a different spectrum of light absorption (∼450 nm) | No effect on SpO 2 readings |
Fetal Hemoglobin | Absorbances of red and near-IR light by fetal hemoglobin (HbF) is the same as HbA | No effect on SpO 2 readings, but could affect co-oximetry SaO 2 depending on instrument’s settings |
Sickle cell anemia | Vaso-occlusive crisis decreases perfusion; Hemolytic crisis increases COHb | ↓ SpO 2 readings SpO 2 overestimates O 2 Hb |
Recent studies have demonstrated a differential bias (formally defined later) depending on participant race, but the mechanism for the differences is still unclear. Skin pigmentation related concerns for pulse oximetry could physiologically be explained if melanin or other optical features of a darker skin in certain racial groups could interfere with the absorption and transmission patterns of oxyhemoglobin and deoxyhemoglobin, therefore altering the absorption ratios . While uncovering the intricacies of these optical interactions with human tissues needs further research, large cohort studies in adults have highlighted greater pulse oximeter inaccuracy and undetected hypoxia in racial groups with dark skin. As a result, regulatory bodies have prioritized the need to study this systematic inaccuracy . Current FDA guidance recommends that device manufacturers conduct a clinical study of 10 or more subjects who vary in age and gender (minimum of 200 data points, paired observations: pulse oximeter, co-oximeter) when evaluating pulse oximeter performance . They also recommend that at least two subjects, or 15 % of the sample (whichever number is greater), should be “darkly pigmented,” because skin tone is understood to impact performance. Knowledge of the statistical output (as discussed below) in pulse oximeter validation studies will enable understanding of how pulse oximeters could meet validation criteria while still outputting biased readings in dark pigmented individuals.
Quantification of pulse oximeter validity
Pulse oximetry validation studies assess Mean Bias, Precision and Accuracy root mean square (ARMS) compared to SaO 2 measurements . With SaO 2 as gold standard, typically SpO 2 values are plotted against a range of SaO 2 measures from 70 to 100 % under controlled settings in healthy adult volunteers breathing air-nitrogen mixtures of varying inhaled oxygen concentrations.
- °
Bias is defined as SpO 2 -SaO 2 for a given pair of measurements in a subject . Mean bias is the average difference between SaO 2 and SpO 2 across the entire dataset and is calculated using the formula: <SPAN role=presentation tabIndex=0 id=MathJax-Element-7-Frame class=MathJax style="POSITION: relative" data-mathml='Bias=∑i=1nSpO2-SaO2n’>Bias=∑𝑛𝑖=1SpO2−SaO2𝑛Bias=∑i=1nSpO2-SaO2n
Bias = ∑ i = 1 n SpO 2 – SaO 2 n
. Therefore, even though the mean bias remains the same across a test data set ( Fig. 2 , line B), the individual bias varies along the spectrum of SaO 2 , and can be significantly higher at low SaO 2 ranges as shown in Fig. 2 . Bias can vary by device, sensor, SaO 2 range and other factors, and may be a positive (SpO 2 > SaO 2 ) or negative value (SpO 2 < SaO 2 ).
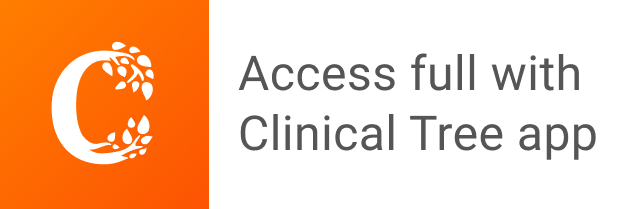