Fig. 12.1
The pulmonary arterial vascular tree. The pulmonary arterial circulation is characterized by a gradual loss of complexity and muscularization from proximal to distal branches of the vascular tree (Townsley 2012). The pulmonary artery is the most proximal and muscularized portion of the arterial circulation. The pulmonary artery consists of defined layers, including the intima (endothelium), the media (smooth muscle), and the adventitia (fibroblasts, microvessels, and vascular precursors). The branching smaller arteries, also referred to as arterioles, are defined by size and muscularization. The small artery/arteriole is composed of 1–2 layers of smooth muscle which completely encircle the endothelium of the vessels. With further branching, smooth muscle becomes less continuous and the most distal vessels and capillaries, consisting of endothelial cells forming a small diameter conduit, are stabilized by a non-continuous layer of pericytes. Pericytes are directly in contact with the endothelium and reside under a continuous basement membrane
Table 12.1
Putative location of stem and progenitor cells in the adult pulmonary vasculature
Artery | Small artery | Distal artery | Alveolar—capillary network | |
---|---|---|---|---|
Characteristics | Muscularized | Muscular arteriole | Non-muscular arteriole | Not muscularized |
1–2 SMC layers | Non-continuous pericyte coverage | Few pericytes with extended processes | ||
Function | Contractile | Contractile | Contractile | Non-contractile |
Associated stem and progenitor cells | Mesoangioblast/EPC, SMC progenitors | SMC progenitors | Pericytes (NG2) | MSC pericytes (ABCG2), EPC |
12.2.2 Mesoangioblasts
The mesoangioblast is a vascular-associated progenitor cell, typically residing in artery wall, that can give rise to vascular cells as well as other mesodermal lineages including skeletal and cardiac muscle. The name “mesoangioblast” distinguishes this cell type from the “hemangioblast,” another progenitor cell that can give rise to vascular as well as hematopoetic lineages. Minasi and colleagues first defined the mesoangioblast by transplanting cells derived from embryonic mouse or quail dorsal aorta into chick embryos (Minasi et al. 2002). Donor cells were found in numerous mesodermal tissues, including bone, smooth, and cardiac muscle. These cells could be cultured and differentiated into osteoblasts, adipocytes, and skeletal myotubes.
Although mesoangioblasts possess a number of cell markers similar to mesenchymal stem cells (MSCs), they cannot be classified as MSCs. Embryonic mesoangioblasts express CD34, while MSCs and pericytes do not (Wang et al. 2012). Mesoangioblasts may also express Flk1, MEF2D, Sca1, and Thy1, with varying reports of cKit (Passman et al. 2008; Campagnolo et al. 2010; Zengin et al. 2006). Transcriptome analysis of mesoangioblasts also determined that they express receptors for FGF, PDGF, TGFβ, and Wnt proteins (Tagliafico et al. 2004).
While mesoangioblasts were first isolated from dorsal aorta, the developmental origin of mesoangioblasts remains unknown. Flk1 lineage tracing determined that Flk1+ positive cells can give rise to skeletal and cardiac muscle, similar to Minasi’s chimeric studies (Motoike et al. 2003). However, Flk1 is not a unique marker for mesoangioblasts. A recent study by Vodyanik and colleagues determined that hESC differentiated to an MSC lineage express genes specific to lateral plate mesoderm during differentiation, indicating that MSC and MSC-like cells have a lateral plate mesodermal origin (Vodyanik et al. 2010). However, the MSC subset Vodyanik studied appeared to possess a more restricted differentiation potential than mesoangioblasts, making it inaccurate to assume that mesoangioblasts arise from lateral plate mesoderm as well.
Mesoangioblasts have been studied as a potential cell therapy source for muscular regeneration, but have not been used to treat pulmonary diseases (Galli et al. 2005; Galvez et al. 2006; Sampaolesi et al. 2003, 2006). Mesoangioblasts were used to treat mice with X-chromosome-linked muscular dystrophy (mdx), alpha-SG-null mice, and golden retrievers with heredity muscular dystrophy. Animals that are administered mesoangioblasts showed some improvement in muscular function. Interestingly, when GFP-labeled mesoangioblasts were injected into the femoral arteries of mice with cardiotoxin-induced muscular injury, 5 % of the injected mesoangioblast population migrated to the lung (Galvez et al. 2006). Whether mesoangioblast treatment could alleviate the pathology of pulmonary injury remains unknown. The role of these cells in remodeling during lung disease has not been evaluated and a precise understanding of their biology is confounded by the lack of a specific marker to define their origin in vivo.
12.2.3 Smooth Muscle Progenitors
Smooth muscle proliferation and hypertrophy are characteristic of vascular remodeling in the pulmonary artery and arterioles. A population of smooth muscle cell (SMC) progenitors is responsible for SMC expansion. These progenitors are hypothesized to reside in tissue interstitium, arterial adventitia as well as the mesothelium. The characterization of smooth muscle progenitors to date includes their identification by cell marker analysis including, but not limited to, smooth muscle alpha actin (acta2), SM22a (tagln), Tie-1, and PDGFRα/β (Majesky et al. 2011b; Minasi et al. 2002). The first smooth muscle progenitors described were defined by their expression of PDGFR α and β during lung development and terminal differentiation and their recruitment to newly forming endothelial tubes by PDGF ligands (Lindahl et al. 1997; Hellstrom et al. 1999).
The mesothelium is an extra-pulmonary tissue that may provide a source of SMC progenitors in the adult lung during tissue homeostasis as well as disease-associated remodeling. The mesothelium is a component of the pleura that encases the adult lung. Mesothelial expression of the Wilms tumor 1 gene (WT1) was exploited to perform lineage tracing of mesothelium to both mesenchymal and smooth muscle lineages within the lung. However, WT-1 expression is not strictly limited to the mesothelial cells in the adult lung, complicating lineage-tracing studies to determine the role these cells play during adult tissue homeostasis and disease.
SMC progenitors in homeostasis, development, and disease are controlled by a variety of cell signaling pathways, including FGF, Shh, Wnt, PDGF, and Notch. FGF10-FGFR2b signaling during lung development prevents SMC differentiation and limits matrix deposition (De Langhe et al. 2006). Studies by Passman et al. demonstrated that a gradient of Shh in the adventitia regulates the number of Sca1 SMC progenitors lacking differentiated markers, but expressing transcription factors of SMC lineage potential, including serum response factor, Klf-4, Msx1, and fox04 (Passman et al. 2008). Regulation of proliferation by the Wnt and PDGFR signaling pathways in SM22-labeled SMC progenitors was also reported by Cohen et al. (2009). A temporal increase in Notch signaling was required for Ve-cadneg/CD45neg/Tie1pos/CD31dim terminal differentiation to SMC, but not the maintenance of a stable vessel (Chang et al. 2012). These complex interactions during development involve coordinated signaling between the epithelium, endothelium, and mesenchyme to regulate appropriate tissue patterning and development.
12.2.4 Pericytes
A pericyte is a perivascular-associated progenitor cell found within the basement membrane of the vasculature. Pericytes were first described by Charles-Marie Benjamen Rouget (Armulik et al. 2011) and named “Rouget cells,” until being renamed for their proximity to endothelial cells by Zimmerman in a 1923 publication. While pericytes are thought to derive from progenitors found in the neurocrest in the forebrain or mesenchyme, lung-resident pericytes also derive from the mesothelium (Que et al. 2008; Bergers and Song 2005). Pericytes, like many mesenchymal progenitor cells, exist in a heterogeneous population with no single cell marker. Also, because many other stromal populations exist in the periendothelial space, they are often confused with other cell types, such as MSC, vascular SMC (vSMC), Fb, and macrophages. Thus, the “gold standard” for pericyte identification is a combination of analysis using multiple markers as well as ultrastructural analysis.
Pericytes have been found to express SMA, Desmin, RGS5, PDGFβ, and NG2 among other markers (Armulik et al. 2011; Crisan et al. 2008; Feng et al. 2010; Rock et al. 2011; Bergers and Song 2005). These makers are not specific to pericytes. For example, all of the markers mentioned are also found on vSMC (Armulik et al. 2011). To correctly identify a pericyte, high-resolution histological examination is needed. Pericytes are vascular associated cells with a large nucleus, small cytoplasm, and long processes encircling the capillary wall (Bergers and Song 2005). Pericytes communicate with endothelial cells through paracrine signaling as well as direct contact in the form of gap and adherens junctions as well as “peg-and-socket” contacts in which pericyte processes are inserted into endothelial invaginations (Armulik et al. 2011; Feng et al. 2010).
Pericytes have been isolated from multiple human organs, including skeletal muscle, placenta, skin, pancreas, and bone marrow (Crisan et al. 2008). Crisan isolated pericytes using flow cytometry based on the expression of CD146 and the exclusion of CD34-, CD45-, and CD56-positive cells. These CD146-positive cells could give rise to adipocytes, chondrocytes, and osteoblasts both in vitro as well as express myofibers when administered to cardioxin-treated mouse skeletal muscle and become ectopic bone when implanted into mouse hind limb (Crisan et al. 2008). Based on marker expression and cell lineage potential, pericytes are similar to MSCs and to date have typically been grouped as the same population due to their perivascular localization.
12.2.5 Mesenchymal Stem Cell
Another potential source of multipotent cells in the lung are the resident MSCs. The first MSCs defined were bone marrow-derived mesenchymal stem cells (BM-MSC) identified by Friedenstein (Friedenstein et al. 1968; Lee et al. 2011). He established several foundational concepts of MSC biology, determining that isolated BM-MSCs grew in tissue culture plates in formations termed colony-forming unit-fibroblast (CFU-F) and that BM-MSCs could differentiate into a host of different mesenchymal lineages. Subsequent work discovered MSC populations outside the BM-MSC, prompting the International Society of Cellular Therapy to define an MSC by three conditions: (1) MSCs be adherent to plastic (2) MSCs must express specific cell markers (CD73, CD90, CD105) but not express other markers (CD45, CD34, CD14, CD11b), and (3) MSCs must differentiate into mesenchymal lineages including osteoblasts, adipocytes, and chondroblasts (Dominici et al. 2006). While it is common to differentiate potential MSC populations to multiple mesenchymal lineages in vitro, such an experiment is difficult to recapitulate in vivo. MSC are distinct from fibroblasts in their ability to form colonies in a CFU-F assay and their previously mentioned multilineage differentiation potential.
MSCs are also prominent in remodeling due to injury. Remodeling in arteries during disease or in response to injury is often coupled with the formation of ectopic tissue structures such as bone, fat, or cartilage as well as microvascularization of the outer most layers (Majesky et al. 2011a). Primitive mesenchymal cells associated with arteries have been termed calcifying vascular cells (CVC) and vascular adventitial fibroblasts (VAFs) and represent an additional group of multipotent mesenchymal stromal cells (MSC) (Tintut et al. 2003; Hoshino et al. 2008). These cells were similar in surface phenotype to bone marrow MSC including the expression of CD44, CD105, and CD29 and lack of all hematopoietic markers as well as CD34, distinguishing these populations from previously described mesoangioblasts (Tintut et al. 2003; Hoshino et al. 2008).
Resident MSC populations identified in the neonate and adult lung described to date differ both in their origin and function (Foronjy and Majka 2012). Lung MSCs have been identified in bronchoalveolar lavage fluid from patient allograft tissue or tracheal aspirates from ventilated neonates, respectively, through their ability to adhere to plastic (Lama et al. 2007; Hennrick et al. 2007). Concurrently, MSC have been identified and isolated by flow cytometry via visualization of a side population phenotype by Hoechst 33342 vital dye staining in combination with the absence of the hematopoietic marker, CD45 (Summer et al. 2007; Martin et al. 2008; Irwin et al. 2007). Lama et al. showed conclusively that MSCs reside in an adult lung in humans by demonstrating donor origin of BAL MSC up to 11 years after transplant (Lama et al. 2007). Jun et al. also showed that MSCs reside in the adult lung by transplanting genetically labeled bone marrow and observing the composition of the MSC following injury in murine models (Jun et al. 2011). Sca1 has also been reported to enrich for a population of mesenchymal/fibroblast progenitor cells resident in lung tissue (McQualter et al. 2009). Multipotent MSC have also been isolated from endarterectomized tissues from patients with chronic thromboembolic pulmonary hypertension (CTEPH) (Firth et al. 2010).
These MSC populations, independent of their origin, demonstrate multilineage mesenchymal differentiation potential to osteocyte, adipocyte, and chondrocyte lineages, and in varying combinations, express the characteristic mesenchymal cell surface determinants ABCG2, CD90, CD105, CD106, CD73, CD44, Stro-1, and Sca1. In addition, they lack the hematopoietic markers c-kit and CD34 (Jun et al. 2011; Martin et al. 2008; Chateauvieux et al. 2007; Summer et al. 2007; McQualter et al. 2009). Both MSC isolated from BAL and via ABCG2 expression are capable of suppressing T-cell proliferation (Jarvinen et al. 2008; Jun et al. 2011; Lee et al. 2009). Analysis of gene expression of lung MSCs compared to BM-MSCs revealed that only lung MSCs express FOXF1, HOXA5, and HOXB5, transcription factors unique to the developing lung (Walker et al. 2011). In addition to delineating BM-MSC from organ-resident MSCs, this finding may indicate that MSCs are inherently organ-specific.
Pericytes and MSC have very similar cell surface characteristics and differentiation potential. Due to these similarities, pericytes have been hypothesized to be MSC in adult tissues. However, the limitations to address this hypothesis included the lack of a specific marker that could be used to distinguish MSC. Pericytes function to stabilize blood vessels, whereas the function of MSC during adult tissue homeostasis, other than as a supporting or stromal cell, is unknown. Recently, studies by Jun and Chow et al. reported the identification of ABCG2 as a marker to label lung MSC, which lack expression of SMA and NG2 protein (Chow et al. 2013; Jun et al. 2011). Further global gene profiling analyses demonstrated that these ABCG2pos lung MSC were distinct from NG2 pericytes as well as lung fibroblasts. The differences between NG2pos pericytes and ABCG2pos MSC likely represent pericyte heterogeneity within the lung. Interestingly, these ABCG2pos MSC exhibit vascular endothelial, smooth muscle, and myofibroblast differentiation potential, additionally qualifying them as endothelial and smooth muscle progenitors (Chow et al. 2013).
To distinguish differences between generic MSC, fibroblasts, side population MSC, pericytes, and ABCG2posMSC, we have formulated a comparison of defined “mesenchymal” cell populations present in the distal lung (Fig. 12.2). Here we compare the initial lung MSC described by the efflux of Hoechst 33342 dye and the appearance of the side population phenotype (CD45neg SP (Summer et al. 2007; Martin et al. 2008; Irwin et al. 2007; Jun et al. 2011)), to lung fibroblasts, pericytes, ABCG2posMSC. All of the populations share common traditional MSC cell surface markers. However, only fibroblasts lack CFU-F ability. Although there is significant overlap, based on global gene expression analysis, the CD45neg SP is a bit broader than the ABCG2posMSC. This is likely due the expression of transporters and additional multidrug-resistant transporters on the cell surfaces of other lung cell populations which can efflux Hoechst dye. This was confirmed by Jun et al. who demonstrated that ABCG2 marked ~80 % of the CD45neg SP (Jun et al. 2011). These findings illustrate that ABCG2 is a more specific marker than the Hoechst 33342 staining. There is significant similarity between the ABCG2posMSC and NG2pos pericytes. ABCG2posMSC are precursors of NG2 pericytes. The differences in gene expression are likely due to function. However, it is clear that in their naïve states, they represent two distinct subpopulations of cells. All of these cell populations share characteristics of the “classically defined MSC.”
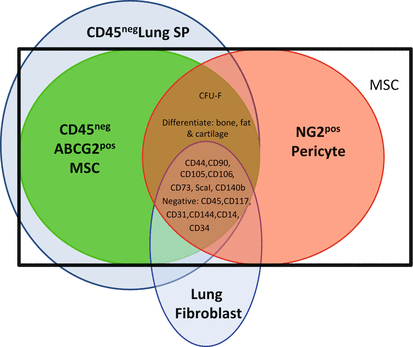
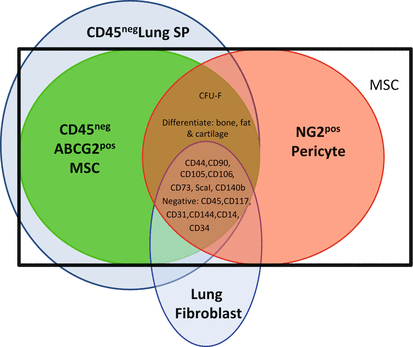
Fig. 12.2
Summary of lung mesenchymal cell subpopulations. Classically defined MSC (black box) have traditionally been isolated based on their ability to adhere to plastic and a panel of cell surface antigens. This limited their study in vivo because fibroblasts and/or endothelial cells express many of the same markers. However, more recently, lung mesenchymal subpopulations have been further delineated by genomic analyses, CFU-F, and multilineage differentiation potential (Chow et al. 2013; Firth et al. 2010; Summer et al. 2007; Jun et al. 2011; Lama et al. 2007; Martin et al. 2008; Irwin et al. 2007). These distinct subpopulations include the CD45neg side population (SP), lung fibroblasts, ABCG2posMSC, and NG2pos pericytes
12.2.6 Endothelial Progenitor Cells and Endothelial Mesenchymal Transition
The endothelial progenitor cell (EPC) is a cell type that generates endothelial cells in response to injury. The first indications of the existence of EPCs came from the spontaneous generation of microvasculature on implanted prosthetics in animals (Scott et al. 1994). Asahara and colleagues were the first to isolate and characterize EPCs from human peripheral blood (Asahara et al. 1997). While Asahara’s initial isolation protocol has been adapted by other researchers (reviewed in Hirschi et al. 2008) (Yoder 2012), the most common method of EPC isolation is to plate peripheral blood on fibronectin-coated dishes, replate non-adherent cells onto separate fibronectin plates to deplete other hematopoietic lineages (such as macrophages and monocytes), and culture cells until clusters emerge at 7 days. Tissue-resident EPCs have also been isolated from artery walls in a location termed “the vasculogenic zone” between the smooth muscle and adventitial layers (Zengin et al. 2006).
While there is no individual accepted marker that defines an EPC, the cells that make up these clusters express CD34, CD133, and VEGFR2/KDR (Hirschi et al. 2008; Mao et al. 2013). Several functional assays define EPCs both in vitro and in vivo. In vitro, EPCs must have the capacity to uptake Dil-Ac-LDL, stain with lectin dye, as well as form tubes when plated in Matrigel (Firth and Yuan 2012). In vivo, EPCs must be able to form tubes when injected into animals in matrigel “plugs.” Several investigators have verified that EPCs contribute to neovascularization in vivo (Asahara et al. 1997; Lam et al. 2008, 2011), and therefore likely have the potential to contribute to pathological remodeling during disease. Studies of the origin, localization, and function of EPC during vascular homeostasis and remodeling are complicated by the lack of tissue and cell-specific markers of this cell type.
Endothelial cells (EC) also represent a potential progenitor population in the vasculature. EC can be induced to become migratory mesenchymal cells through the loss of endothelial markers and the progressive gain of mesenchymal characteristics (Kalluri and Weinberg 2009). Endothelial-to-mesenchymal transition (or EndoMT) has mainly been studied in the context of endocardial cushion and heart valve formation, but has become the focus of many investigators in research into sources of myofibroblasts during fibrosis. EndMT can be induced after bleomycin injury to the lungs (Hashimoto et al. 2010). Sixteen percent of lung fibroblasts isolated from transgenic animals expressing beta-galactosidase in TIE2+ cells treated with bleomycin were lacZ+, indicating these cells were likely derived from a vascular origin, the caveat of these studies being that Tie-2 expression is not restricted to EC.
Endothelial cells may also be induced to exhibit MSC characteristics. Medici and colleagues determined that a mouse model with constitutive activation of Alk2 had endothelially derived chondrocytes and osteoblasts (Medici et al. 2010). Injection of BMP4, an Alk2-activating ligand, could also generate endothelially derived chondrocytes and osteoblasts. Endothelial cell lines transfected with an Alk4-inducing adenovirus also began to show expression of the MSC markers STRO-1, CD10, CD44, CD71, and CD90. In addition, endothelial cells treated with TGFβ2 and BMP4 (both activators of Alk4) and cultured in osteogenic, chondrogenic, and adipogenic medium could differentiate into these three lineages. Endothelial cells treated with TGFβ2 and BMP4, placed in sponges and implanted into nude mice, could also differentiate into bone cartilage or fat. Taken together, these studies demonstrate that endothelial cells can acquire MSC phenotypes both in vivo and in vitro.
12.3 The Role of Resident Lung Stem Cells and Progenitors in Vascular Remodeling
12.3.1 Cell-Based Mechanisms of Vascular Remodeling During Lung Disease
Many of the cellular changes associated with PH include an increase in myofibroblasts expressing SMA (Morrell et al. 2009). The resident lung origin of these cells is hypothesized to be vascular SMC or adventitial fibroblasts. More recently, the possibility of resident progenitor cells or the process of endothelial-to-mesenchymal transition (EndoMT) is considered. Existing studies supporting a role for the contribution of resident lung progenitor and stem cells to pulmonary remodeling are summarized. To our knowledge, mesoangioblasts have not been studied in pulmonary remodeling and so are not included.
12.3.2 Smooth Muscle Progenitors
Excessive accumulation of SMCs and collagen in the vasculature is common to many adult lung diseases including PAH, PH associated with ILD or COPD, and lymphangioleiomyomatosis (LAM) (Ferri et al. 2004; Benedict et al. 2007; Hansmann et al. 2008; Harrison et al. 2005; Hemnes et al. 2011; Hong et al. 2008; Humbert et al. 2004; Majka et al. 2008, 2011; Rubin and Galie 2004). That being said, studies assessing the contribution of smooth muscle progenitors to vascular remodeling during adult lung disease are rather limited. Cohen et al. defined Wnt regulation of SM22 expressing SMC progenitor proliferation and differentiation during development through a tenascin C-PDGFR pathway (Cohen et al. 2009). The PDGFR pathway was also upregulated in vascular smooth muscle from pulmonary hypertension patients relative to controls. These studies illustrate the reactivation of developmental pathways during adult disease.
12.3.3 Pericytes
Pericytes have been implicated in vascular pathology, but there is some debate into the role they play in the process of lung remodeling. Pericytes function to stabilize vasculature and embryos lacking pericyte coverage are not viable (Armulik et al. 2011; Bergers and Song 2005). Whether the proliferation by and increased muscularization of arterioles by pericytes causes adaptive or adverse remodeling is a topic of current debate. Pericytes are also known to play a substantial role in the genesis of myofibroblasts and adverse remodeling associated with kidney fibrosis (Kida and Duffield 2011; Lin et al. 2008).
Rock and colleagues employed lineage tracing of NG2-expressing pericytes and determined that cells positive for PDGFRβ, desmin, and NG2 proliferated 21 days after bleomycin injury, but that the NG2+ cells do not substantially differentiate into αSMA+ myofibroblasts. This data implies that while NG2+ pericytes expand in response to injury, they do not become myoFB. However, a recent study by Ricard et al. demonstrated that not only do NG2+ cells expand in numbers 21 days post-hypoxia injury, but that the population of NG2+/SMA+ cells doubles in number as well (Ricard et al. 2014). While the proliferative response of pericytes to lung injury is becoming recognized, the role they play in disease is still relatively unknown.
Pericytes have been shown to play a role in some vascular diseases. Abnormal pericyte coverage of vessels has been implicated as causative for pulmonary hypertension associated with Adams–Oliver syndrome. Adams–Oliver syndrome features capillary rarefaction, loss or hyperproliferation for capillaries, and vessel stenosis (Patel et al. 2004). Loss of capillaries and vasculopathy are also major contributors to clinical manifestations of scleroderma (Fleming et al. 2009). However, Fleming et al. demonstrated that high dose immunosuppressive and hematopoietic stem cell therapies reversed the loss of functional pericytes, decreased rgs5 expression, and induced capillary regeneration (Fleming et al. 2008). These data suggest that preserving the function of pericytes and vascular supporting cells may have implications for preventing the progression of PAH or pulmonary hypertension associated with other chronic lung disease.
12.3.4 Mesenchymal Stem Cell
Mesenchymal cell proliferation and apoptosis are factors that determine whether a fibroproliferative response resolves, as in wound healing, or progresses to a chronic pathologic condition, as in pulmonary hypertension and fibrosis (Bonner 2010).
Functional studies of the ABCG2pos Hoechst33342dim CD45neg-resident lung MSCs demonstrate that they regulate the severity of bleomycin injury via modulation of the T-cell response (Jun et al. 2011). Jun et al. elegantly documented that bleomycin treatment of mice induced the loss of these endogenous lung MSCs and elicited fibrosis (Cui et al.), inflammation, and PAH. Replacement of resident stem cells by administration of isolated lung MSCs attenuated the bleomycin-associated pathology and mitigated the development of PAH. These data suggest that lung MSCs function to protect lung integrity following injury; however, when endogenous MSCs are lost, this function is compromised. Lung MSCs may therefore regulate homeostasis and repair of their native tissue, the distal lung.
In addition to their reparative properties, several studies indicate that lung MSCs under certain conditions mediate pathogenic changes within the lung (Bonner 2010; Pierro and Thebaud 2010). Firth et al. described the isolation of MSC from endarterectomized tissues from patients with CTEPH (Firth et al. 2010). The isolation of both myofibroblasts and functional MSC fibrotic coagulant tissue suggested that multipotent lung MSC might participate in the vascular remodeling associated with pulmonary hypertension. More recently, lineage-tracing analyses performed by Chow and colleagues confirmed the lineage potential of lung MSC in vivo. Using an inducible ABCG2-driven expression of Cre and an eGFP label, these studies demonstrated that multipotent MSC contributed to muscularization of distal lung microvessels in a murine model of hypobaric hypoxia-induced PAH (Chow et al. 2013). When these cells were genetically depleted of superoxide dismutase, a potent antioxidant, their contribution to muscularization was significantly increased in the murine model of PAH. In vitro, increased oxidant stress resulted in transition of the MSC to a contractile myofibroblast phenotype. This transition from a MSC to a contractile myofibroblast was correlated to alterations in Wnt pathway signaling.
Indeed, the behavior of MSCs is highly sensitive to the microenvironment to which these cells are exposed (Yan et al. 2007). As an example, it was recently shown that TGF-β expression within the lungs of premature infants stimulates MSCs to differentiate into myofibroblasts (Popova et al. 2010). Myofibroblasts induce abnormal matrix remodeling that results in the development of the chronic lung disease, BPD. BPD is characterized by decreased functionality of the alveolar-capillary surfaced for gas exchange including both the vasculature and airways (Toti et al. 1997). Similar findings were observed in lung allografts from transplanted patients (Walker et al. 2011). In this study, lung-derived MSCs isolated from the airways had increased expression of type I collagen and α-smooth muscle actin and readily differentiated into myofibroblasts upon treatment with IL-13 or TGF-β (Walker et al. 2011). Allograft survival is limited by bronchiolitis obliterans (BOS), a fibrotic constriction of the airways (Todd and Palmer 2011). MSCs from BOS subjects had a profibrotic phenotype, indicating that this cell type was an important mediator of the fibrotic changes and associated remodeling. Thus, these findings indicate that MSCs are a critical factor in the development of dysfunctional lung vascular and interstitial remodeling in these adult lung diseases.
12.3.5 EPC and EndoMT
EPCs, while difficult to define conclusively, have been studied as an indicator of disease as well as a potential treatment for neonatal lung disease. Heterogeneity within EC populations and lack of a specific definitive marker (Rajotte et al. 1998) has limited our understanding of the true differentiation potential of resident lung progenitor populations. Typically, tissue-resident EPC share markers with differentiated ECs and in some cases MSC (along with hematopoietic and circulating cells). These common markers include VE-cadherin (CD144), Tie-1/2, VEGFR1/2, and PECAM (CD31). EPC levels in the peripheral circulation of patients with pulmonary disease have been correlated to their prognosis and severity (Burnham et al. 2005). Recently, Alphonse and colleagues were able to isolate a subset of EPCs known as endothelial colony-forming cells (ECFCs) from human fetal lung (Alphonse et al. 2014). These EPCs could be altered by hypoxic injury to form fewer colonies in vitro as well as fewer capillary networks in capillary assays. In addition, ECFCs isolated from a rat model of BPD had similar reductions of functionality. However, human umbilical cord ECFC delivered through intra-jugular injections to the BPD rat-model preserved alveolar architecture, vasculature, and reduced hypoxic-related PH, showing for the first time that EPCs can be used to treat the alveolar as well as vascular abnormalities associated with BPD.
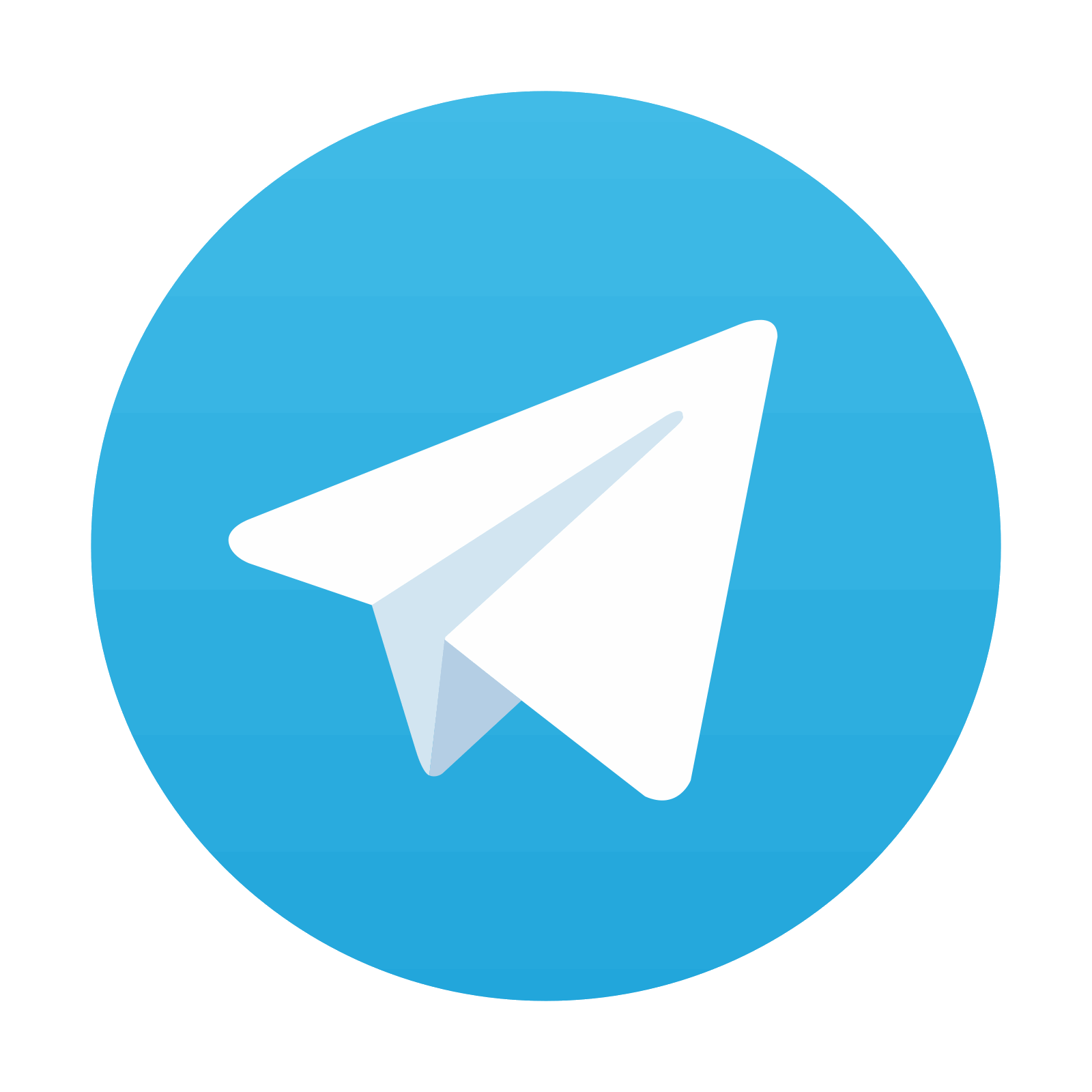
Stay updated, free articles. Join our Telegram channel
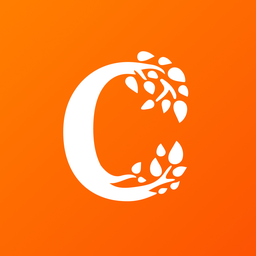
Full access? Get Clinical Tree
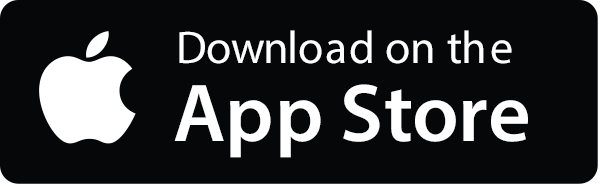
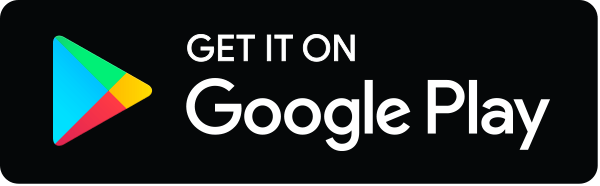