Chapter 29 Pulmonary vascular disease


Pulmonary Oedema
Anatomical Factors
The pulmonary capillary endothelial cells abut against one another at fairly loose junctions which are of the order of 5 nm wide.1 These junctions permit the passage of quite large molecules and the pulmonary lymph contains albumin at about half the concentration in plasma. Alveolar epithelial cells are connected by tight junctions at their alveolar surface with a gap of only about 1 nm. Under normal circumstances the tightness of these junctions prevents the escape of large molecules, such as albumin, from the interstitial fluid into the alveoli. However, the proteins that make up the tight junction are not simply passive structural units, and can, for example in response to nitric oxide, be modified and allow an increase in permeability across the tight junction.2,3
The lung has a well-developed lymphatic system draining the interstitial tissue through a network of channels around the bronchi and pulmonary vessels towards the hilum. Lymphatic vessels are seen in the juxtaseptal alveolar region (see below) and are commonly found in association with bronchioles. Down to airway generation 11 (see Table 2.1), the lymphatics lie in a potential space around the air passages and vessels, separating them from the lung parenchyma. In the hilum of the lung, the lymphatic drainage passes through several groups of tracheobronchial lymph glands, where they receive tributaries from the superficial subpleural plexus. Most of the lymph from the left lung usually enters the thoracic duct where it can be conveniently sampled in animals. The right side drains into the right lymphatic duct.
The normal lymphatic drainage from human lungs is astonishingly small – only about 10 ml per hour. However, lymphatic flow can increase up to ten times this value when transudation into the interstitial spaces is increased.4 This presumably occurs when pulmonary oedema is threatened but it cannot be conveniently measured in man.
Pulmonary Fluid Dynamics
For intravascular fluid to enter the alveoli it must traverse three barriers. First, it must move from the microcirculation into the interstitial space (across the endothelium), secondly through the interstitium and finally from the interstitial space into the alveoli (across the epithelium) (Figure 29.1).
![]() | is the flow rate of transudated fluid which, in equilibrium, will be equal to the lymphatic drainage. |
K | is the hydraulic conductance (i.e. flow rate of fluid per unit pressure gradient across the endothelium). |
Pcap | is the hydrostatic pressure in the pulmonary capillary. |
Pint | is the hydrostatic pressure in the interstitium. |
Σ | is the reflection coefficient, in this case applying to albumin. It is an expression of the permeability of the endothelium to the solute (albumin). A value of unity indicates total reflection corresponding to zero concentration of the solute in the interstitial fluid. A value of zero indicates free passage of the solute across the membrane and, with equal concentrations on both sides of the membrane, the solute could exert no osmotic pressure across the membrane. This normally applies to the crystalloids in plasma. |
![]() | is the osmotic pressure the solute exerts within the pulmonary capillary. |
![]() | is the osmotic pressure the solute exerts in the interstitium. |
Under normal circumstances in humans, the pulmonary lymph flow is about 10 ml per hour with a protein content about half that of plasma. The pulmonary microvascular pressure (Pcap) is in the range 0–2 kPa (0–15 mmHg), relative to atmosphere, depending on the vertical height in the lung field. Furthermore, there is a progressive decrease in capillary pressure from its arterial to its venous end, since approximately one-half the pulmonary vascular resistance is across the capillary bed (see Figures 7.2 and 29.1). In this context, it is meaningless to think of a single value for the mean pulmonary capillary pressure.
The hydrostatic pressure in the interstitial space (Pint) of the lung is not easy to measure. Animal studies using micropuncture techniques obtained subatmospheric pressures of −0.40 to −1.25 kPa (−4 to −12.5 cmH2O).6 In the excised lung there was no vertical gradient in interstitial pressures such as might have been expected from the effect of gravity, but this was observed when measurements were made with the chest and pleura intact.6
The reflection coefficient for albumin (Σ) in the healthy lung is about 0.5. The overall osmotic pressure gradient between blood and interstitial fluid is about 1.5 kPa (11.5 mmHg). Thus there is a fine balance between forces favouring and opposing transudation. There is a considerable safety marginin the upper part of the lung where the capillary hydrostatic pressure is lowest. However, in the dependent part of the lung, where the hydrostatic pressure is highest, the safety margin is slender.
Fluid dynamics within the interstitium. It is now accepted that the interstitium does not simply act as a passive conduit for fluid transfer to the lymphatics.7,8 Proteoglycan and hyaluron molecules are present in the pulmonary interstitium of animals, and function like a gel to absorb water to minimise increase in interstitial pressure and prevent hydration of other extracellular structures such as collagen.9 Regional differences in the properties of these molecules are believed to be responsible for the establishment of a pressure gradient between the septal interstitium and the juxtaseptal region where lymphatic channels originate. This gradient may promote, and allow some control of, fluid flow from the endothelium to the lymphatics in the normal lung.7
With increased fluid transfer across the endothelium, the interstitial space can accommodate large volumes of water with only small increases in pressure, the interstitial compliance being high. Some 500 ml can be accommodated in the interstitium and lymphatics of the human lungs with a rise of pressure of only about 0.2 kPa (2 cmH2O).5 Eventually, the capacity of the molecules to absorb water is exceeded, and the proteoglycan structure breaks down, possibly leading to disturbances of nearby collagen molecules and therefore basement membrane function, producing alveolar oedema.7 Alterations of interstitial proteoglycan structure during lung injury may contribute to the greater likelihood of pulmonary oedema under these circumstances (Chapter 31).
Fluid exchange across the alveolar epithelium. The permeability of this barrier to gases is considered in Chapter 9. It is freely permeable to gases, water and hydrophobic substances but virtually impermeable to albumin.
There is now considerable evidence of active fluid clearance from the alveoli in normal human lungs.3,10,11 For methodological reasons, most studies of this system have involved Type II alveolar epithelial cells, but the same processes are believed to occur in Type I cells and in clara cells in the distal airways. On the alveolar side of these cells, the cell membrane contains epithelial sodium channels (ENaC)12 and cystic fibrosis transmembrane conductance regulator (CFTR) channels (page 413), which actively pump sodium and chloride ions respectively into the cell.3 On the interstitial border of the cells, chloride moves passively out of the cell and the Na+/K+-ATPase channel actively removes sodium from the cell. Water from the alveolus follows these ion transfers down an osmotic gradient into the interstitium. Aquaporins are found in human alveolar epithelial cells, suggesting that water movement may be facilitated by these water channel proteins, but their role in normal adult lung remains unclear.3
A small amount of active clearance of fluid from the alveoli occurs under normal circumstances, but these systems become vital when pulmonary oedema threatens. Active removal of alveolar fluid by alveolar epithelial cells increases within one hour of the onset of oedema.13 Stimulation of β2 adrenoceptors by catecholamines increases the affinity of existing Na+/K+-ATPase channels for sodium and causes new channels to be incorporated into the cell membrane from intracellular endosomal stores. After a few hours, a variety of hormones13 (e.g. thyroxine, aldosterone, glucocorticoids) and cytokines3,11 (e.g. tumour necrosis factor) induce the transcription of new Na+/K+-ATPase channels and increase fluid clearance. These mechanisms are important both for minimising the severity of pulmonary oedema and clearing oedema fluid once the precipitating cause has resolved.
Stages of Pulmonary Oedema
Stage I. Interstitial pulmonary oedema. In its mildest form, there is an increase in interstitial fluid but without passage of oedema fluid into the alveoli. With the light microscope this is first detected as cuffs of distended lymphatics, typically ‘8’-shaped around the adjacent branches of the bronchi and pulmonary artery (Figure 29.2). Electron microscopy shows fluid accumulation in the alveolar septa but this is characteristically confined to the ‘service’ side of the pulmonary capillary which contains the stroma, leaving the geometry of the ‘active’ side unchanged (see page 21 and Figure 2.7). Thus, gas exchange is better preserved than might be expected from the overall increase in lung water. Interstitial swelling is however not without risks, and swelling on the service side will eventually cause narrowing of the capillary lumen, though this does not occur until pulmonary oedema is advanced.
Stage II. Crescentic filling of the alveoli. With further increase in extravascular lung water, interstitial oedema of the alveolar septa is increased and fluid begins to pass into some alveolar lumina. It first appears as crescents in the angles between adjacent septa, at least in lungs which have been fixed in inflation (Figure 29.2). The centre of the alveoli and most of the alveolar walls remain clear, and gas exchange is not grossly abnormal, but dyspnoea at rest is likely and the characteristic butterfly shadow may be visible on the chest radiograph.
Stage III. Alveolar flooding. In the third stage, there is quantal alveolar flooding. Some alveoli are totally flooded while others, frequently adjacent, have only the crescentic filling or else no fluid at all in their lumina. It seems that fluid accumulates up to a point at which a critical radius of curvature results in surface tension sharply increasing the transudation pressure gradient. This produces flooding on an all-or-none basis for each individual alveolus. Due to the effect of gravity on pulmonary vascular pressures (page 123), alveolar flooding tends to occur in the dependent parts of the lungs. Râles can be heard during inspiration and the lung fields show an overall opacity superimposed on the butterfly shadow.
Clearly there can be no effective gas exchange in the capillaries of an alveolar septum which is flooded on both sides, and blood flow through these alveoli constitutes venous admixture or shunt. This results in an increased alveolar/arterial Po2 gradient and hypoxaemia, which may be life threatening. Blood flow to the oedematous lung regions is slightly reduced by hypoxic pulmonary vasoconstriction (page 108), possibly in conjunction with interstitial swelling causing capillary narrowing (see above), but the shunt commonly remains substantial.
Hypercapnia is not generally a problem. In less severe pulmonary oedema, there is usually an increased respiratory drive, due partly to hypoxaemia and partly to stimulation of J receptors (page 68). As a result the Pco2 is usually normal or somewhat decreased.
Stage IV. Froth in the air passages. When alveolar flooding is extreme, the air passages become blocked with froth, which moves to and fro with breathing. This effectively stops all gas exchange and is rapidly fatal unless treated.
Aetiology of Pulmonary Oedema
Increased capillary pressure (haemodynamic pulmonary oedema). This group comprises the commonest causes of pulmonary oedema. There is an elevation of the hydrostatic pressure gradient across the pulmonary capillary wall, until it exceeds the osmotic pressure of the plasma proteins. Interstitial fluid accumulates until it overwhelms the ability of the interstitium to absorb fluid and transport it to the lymphatics. Fluid then begins to enter the alveoli and will initially be actively removed by the alveolar epithelial cells until this system is also overwhelmed. The oedema fluid has a protein content which is less than that of normal pulmonary lymph or plasma.5 Apart from transudation in accord with the Starling equation, severe pulmonary capillary hypertension may result in loss of structural integrity (see below).
Causes of an increase in pulmonary capillary pressure are numerous:
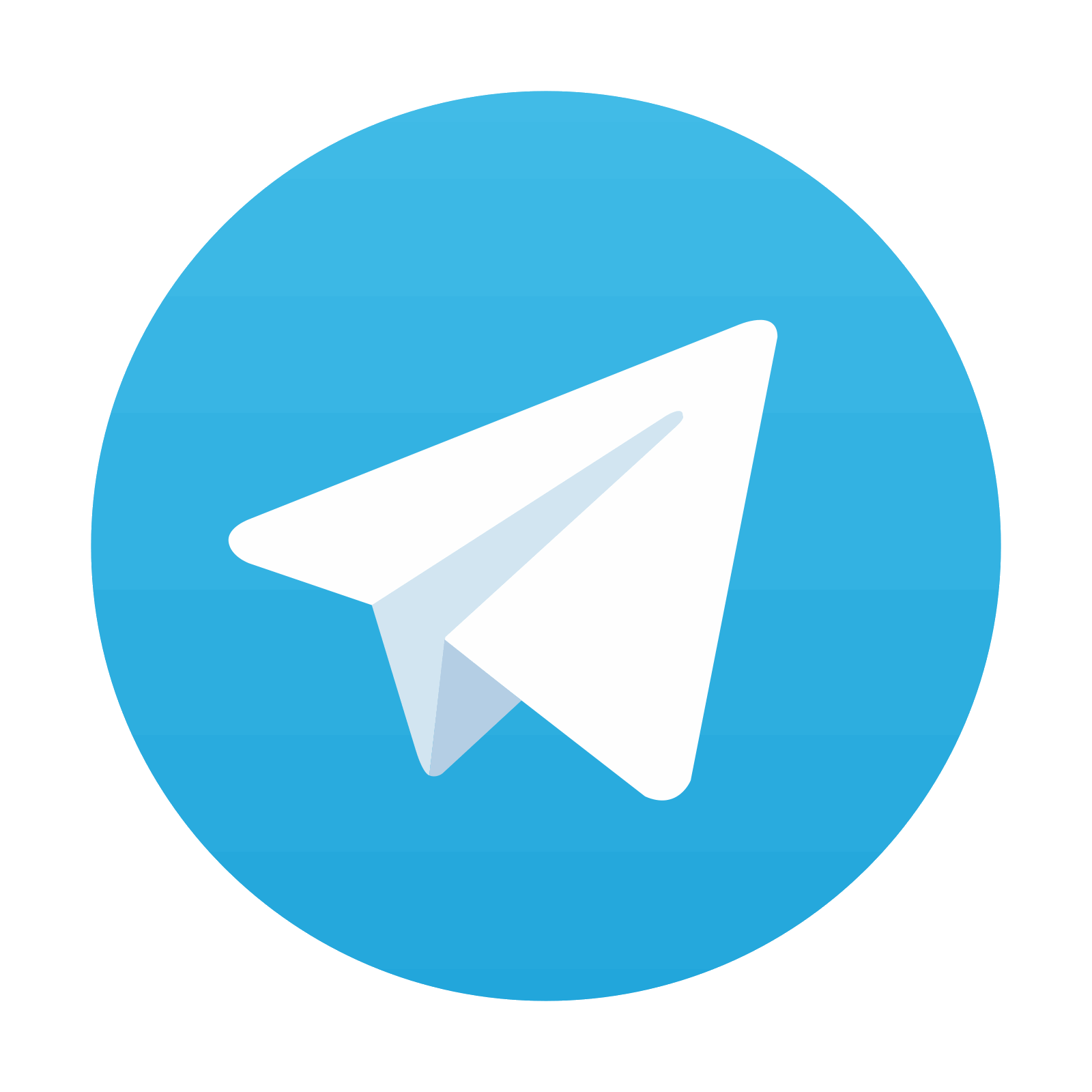
Stay updated, free articles. Join our Telegram channel
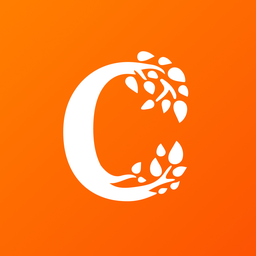
Full access? Get Clinical Tree
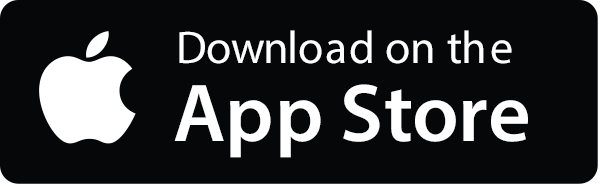
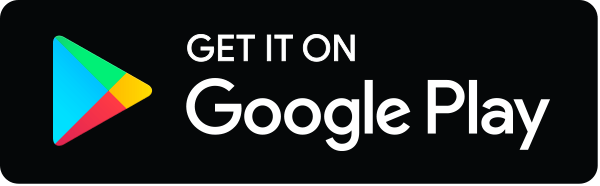