Casts of the airway (yellow/white), arterial (red) and venous (blue) systems in the human lung.
The vessels of the pulmonary vasculature are generated during fetal lung development through branching processes. Further development and remodeling occurs after birth during the postnatal phase of lung morphogenesis and also as the lungs grow throughout childhood to match and support the increase in size of the body. This chapter will focus on the patterning and development of the arterial, capillary, venous, and lymphatic systems in the lung as well as the main pulmonary arteries and veins that connect the heart to the lung. Current knowledge of cellular and molecular pathways that orchestrate and regulate vascular morphogenesis will be discussed. Coordinate regulation of the pulmonary vascular system with airway development will also be introduced. Detailed information on the stages and regulation of airway morphogenesis is presented in Chapter 2 and will not be repeated here. For a long period of time the main period of pulmonary vascular development was believed to occur principally during the third canalicular stage of lung morphogenesis. As will be discussed later in this chapter, with transgenic mice that utilize endothelial specific markers (1) vascular morphogenesis actually starts much earlier in the embryonic phase as the lung buds develop from the foregut endoderm.
Before discussing pulmonary vascular development, it is important to understand the structure of the mature vascular system that is generated. The pulmonary arterial system in the adult lung is highly extensive and extremely complex and for the most part mirrors the airway system (Figure 3-1). The venous and lymphatic systems are similarly complex but have different patterning and locations within the lung. While the airways conduct air in and out through the same system, the vascular system conducts blood in and out through different systems with the arterial system bringing deoxygenated blood from the right side of the heart to the alveolar capillary surface and the venous system returning oxygenated blood to the left. Like the airways, the vascular systems are highly stereotyped and have similar branching patterns from individual to individual, indicating that regulation of the patterning of these systems is robust and reproducible (Figure 3-2). The development of the pulmonary vascular system is closely coordinated with the airways. Genes and molecular pathways that play a role in airway epithelial to mesenchymal signaling are discussed later in this chapter as each of the vascular systems is reviewed. The geometry of the airway and vascular systems are tree-like in structure (Figures 3-1 and 3-2) and are proposed to be fractal in nature, so their generation is likely governed by simple recursive rules, probably involves simple iterative processes, and possibly involves the interaction of only a few pathways and genes/proteins (2).
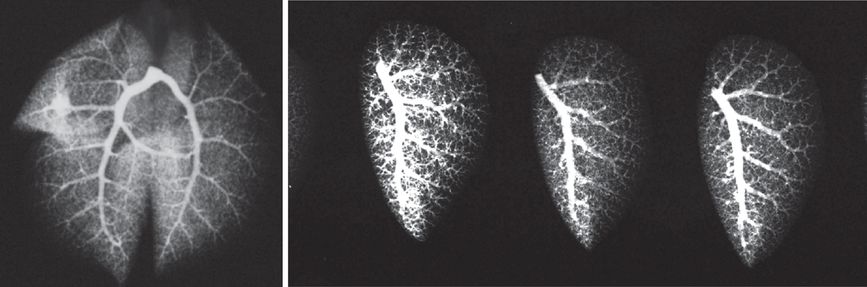
Arteriogram of the whole adult mouse lung (left panel). Arteriograms of left lungs from three adult mice (right panel). Barium mixed with gelatin were instilled into the main pulmonary artery and the lungs inflation fixed. X-ray images were then obtained.
In addition to the complex three-dimensional branching structure of the pulmonary circulation, the caliber and vessel wall structure varies considerably depending on the size, location, and to which system the vessel belongs. The tapering caliber and branching pattern of the arterial system in the adult mouse lung is shown in Figure 3-2. The diameter of the pulmonary arteries varies considerably from proximal to distal arteries. The cellular and molecular structure of the vessel wall as well as the vessel patterning must be carefully regulated so that hemodynamic forces are accommodated, especially because large changes in hemodynamics occur with exercise. In addition to regulation of branching patterns of the vascular and airway systems and the structural changes in the walls of these systems, the developing lung must fit within the constraints of the chest and be connected to the heart and upper respiratory tract, respectively.
Development of the Pulmonary Arteries
Structure, Origins, Timing, and Patterning
It is widely accepted that the airways act as a template for vascular morphogenesis in the lung, especially as the developing arteries align with the conducting airways as they undergo branching morphogenesis. The timing and naming of the stages of lung morphogenesis relied heavily on the histologic appearance of the developing airways. Hislop (3) developed a matching scheme outlining the timing and stages of pulmonary vascular development relative to airway morphogenesis (Figure 3-3). The arteries that run alongside the conducting airways are referred to as conventional arteries and extend out to the respiratory bronchioles and alveolar ducts (3). Supernumerary arteries arise from the conventional arteries and lead in a direction away from the conducting airways to supply the alveolar regions (Figure 3-4). The main (extra) pulmonary arteries and veins that connect the heart to the pulmonary circulation were originally thought to develop by angiogenesis, sprouting off the heart and growing into the developing lungs (4). However, there is also evidence that they may form by vasculogenesis, as had already been reported for the aorta (5). Further discussion of this occurs later in the chapter.
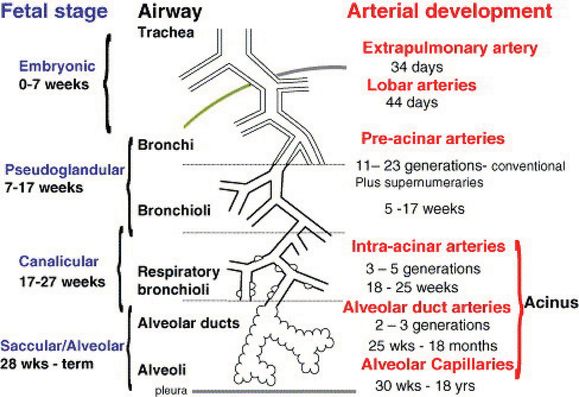
Stages and timing of airway and arterial development in humans.
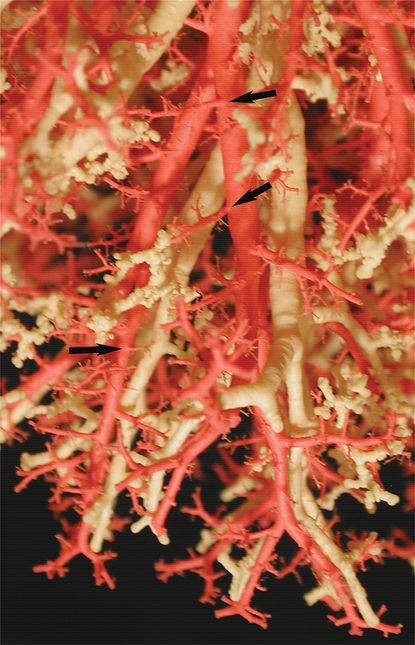
Casts of the airway (yellow/white) & arterial (red) systems in the human lung shows close coordination between airway and arterial structure. Arrows indicate supernumerary pulmonary arteries coming off the main conventional arteries and supplying the alveolar regions.
Two major processes are believed to account for the development of pulmonary vessels. One is vasculogenesis, the de novo formation of vessels by differentiation of mesoderm progenitor cells into endothelial cells. Expression of specific genes, including VEGFR2 (Flk-1), CD31 (PECAM), and Sox-17 (see later), defines these cells as well as their location and the structures they form. Studies suggest that early/primitive endothelial cells come together to form primitive tubes or sinusoids. The second process by which vessels are formed is called angiogenesis. In this process new vessels are formed by branching or sprouting from a preexisting vessel. Angiogenesis still occurs in adult organs (although vasculogenesis is not believed to occur) and is one of the key processes that permits tumors to grow. However, it is uncertain if vasculogenesis occurs outside the periods of organogenesis. The relative contribution of these two processes to pulmonary vascular development and the timing has been the cause of some debate. Early attempts to answer these questions in the mouse lung were approached using a variety of injection techniques to generate vascular casts and angiograms as well as histology (4). DeMello and colleagues (4) suggested that vasculogenesis occurs in the peripheral lung to form blood lakes and that angiogenesis is the primary process in the proximal lung that forms the main arteries and veins. They also reported that branching of the airways and vessels is relatively synchronized, although branching of the vasculature is slightly behind the airways. Using their injection techniques, they did not detect fusion between the developing central and peripheral vascular systems until E13–14 in the mouse, and only by E17 was an extensive network present. The concept of peripheral vasculogenesis generating the distal vessels and central angiogenesis the main vessels developed from this report (6).
The identification of genes and molecular markers of early endothelial cells enabled transgenic reporter mice to be developed to facilitate the characterization of pulmonary vascular development. The VEGF receptor 2 (VEGFR2) also known as fetal liver kinase1 (Flk) is an early marker of endothelial cells and is required for endothelial cell differentiation and vasculogenesis. Schachtner and colleagues (1) used transgenic mice with the β-galactosidase gene (LacZ) knocked into the Flk gene locus to identify newly differentiating endothelial cells in the embryonic mouse lung. Their analysis started at E10.5, soon after the lung buds appear, and then continued the analysis through pre- and postnatal development and into the adult lung. Their study demonstrated that vascular development occurs at the earliest stages of lung development, in the embryonic phase as the lung buds first develop. A primitive vascular plexus or “halo” of differentiating endothelial cells formed around each airway bud as it emerged into the underlying splanchnic mesenchyme. In addition to the primitive vascular plexus forming around the airway buds, they also showed that the main pulmonary artery was already forming at E10.5 and had at least a partial lumen. This was much earlier than previously thought, and the developing pulmonary artery was already contiguous with the aortic sac of the heart at E10.5. Because differentiating endothelial cells forming the pulmonary artery could already be seen prior to the formation of a complete lumen, Schachtner and colleagues (1) further suggested that vasculogenesis is the principal mechanism by which proximal arteries are formed, rather than angiogenesis, similar to the aorta that also forms initially by vasculogenesis.
The study by Schachtner et al. (1) highlighted three important concepts in pulmonary vascular development: First, vasculogenesis is the initial process giving rise to the main pulmonary artery that connects the heart to the lung. Second, vascular development in the lung occurs early as the lung bud emerges and so is already well underway by the canalicular stage of lung morphogenesis. Third, pulmonary vascular development continues through all stages of lung morphogenesis. However, the relative contribution of vasculogenesis versus angiogenesis to pulmonary vascular development is still not conclusively resolved, and a third mechanism of new vessel formation has also been proposed: Intussusceptive angiogenesis is a process by which new vessels are generated from existing vessels through the formation of tissue pillars that divide or split the vessel (7). The role of this process in pulmonary vascular development is unclear.
As lung morphogenesis proceeds from E10.5–13.5 in the mouse and the lung buds continue to branch into the splanchnic mesenchyme, extensive endothelial plexuses form around each epithelial bud, including apparent lumens that contain red blood cells (1) (Figure 3-5). Extension of the vascular plexus continues from the embryonic phase through the end of the pseudoglandular phase. Further analysis of Flk-LacZ mice showed that the distal vascular plexus expands and refines so that by E12.5 and E13.5, an extensive system of channels has formed a few cell layers away from the epithelial tubes (1).
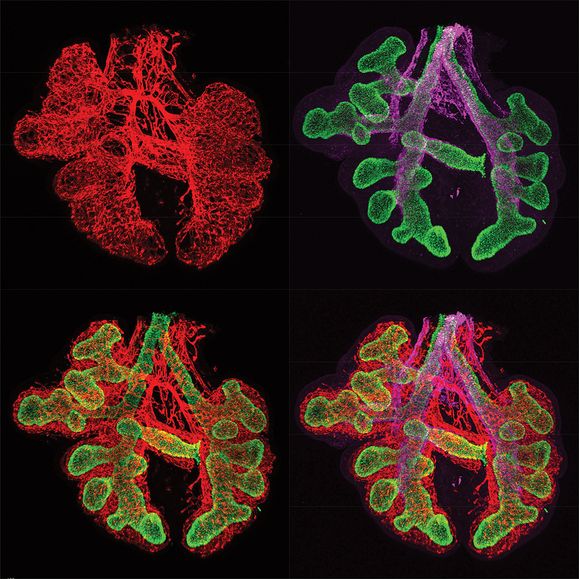
Immunofluorescent staining of fetal mouse lung embryonic day 12.5 (E12.5) showing epithelial cells in green (TTF-1/NKX2.1), endothelial cells in red (endomucin), and smooth muscle cells in purple (smooth muscle α-actin).
Immunostaining for Sox-17, one of the Sox family of transcription factors, also suggests that the process of vasculogenesis plays an important role in the distal lung mesenchyme. Sox-17 marks the differentiation of primitive cells in the mesenchyme into endothelial cells at E12.5 that have not yet formed a lumen (Figure 3-6). In addition, Sox17 expression is also in endothelial cells that have formed primitive tubes and contain red blood cells. In Figure 3-6, showing further immunostaining for Sox17, endothelial cells align with and form vessels with lumens around the developing airways. From E12.5–15.5 the vascular network becomes increasingly more complex and organized with a circulation already present, including veins. By the end of the pseudoglandular phase, all the preacinar arteries and veins have formed (3). The saccular and alveolar phases of lung morphogenesis are characterized by extensive changes in the terminal airways to give rise to alveolar ducts and alveoli, the gas exchange structures of the lung. In parallel there is extensive development of a capillary bed in the distal lung and around the alveoli. Once again this was beautifully shown in the Flk-LacZ mice (1). These later stages are also characterized by thinning of the mesenchyme and epithelial layers to create the thin interface between distal airways and the capillary system.
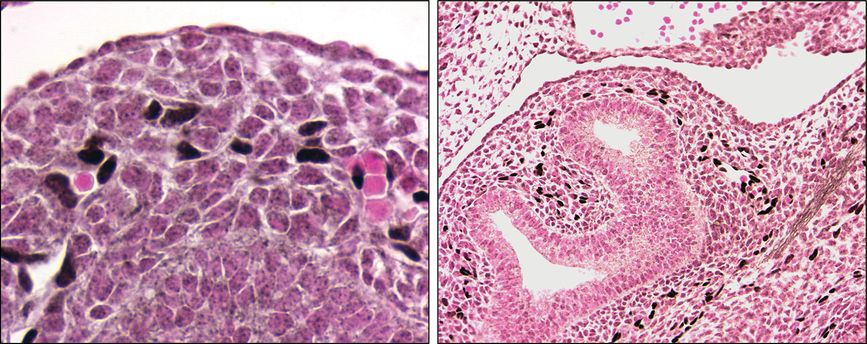
Immunostaining shows differentiation of mesenchymal cells into Sox-17 positive endothelial cells (black) in the mouse lung at embryonic day 12.5 (E12.5). Left panel shows a high-power picture of Sox-17 positive cells (black nuclei) where no patent vessel appears to be present, as well as Sox-17 positive cells, where small vessels contain red blood cells. Right panel shows a lower-power image of E12.5 mouse lung with the location of Sox-17 positive cells (black) in the mesenchyme around the developing airway in the center.
After formation of a primary vascular plexus, the patterning of the arteries, veins, and lymphatics is generally thought to occur in a proximal to distal fashion. One of the best examples of this is the recruitment of smooth muscle around the arteries (and airways) first proximally but then extending more distally as lung development proceeds. These developing vessels also remodel, laying down a vital extracellular matrix in very coordinated layers and with an exact composition. This secondary stage in vascular morphogenesis is vital, as if it is not done correctly, vascular leak and/or hemorrhage can result. Depending on the location and diameter of the artery, the variable thickness, and composition of the arterial wall, and there may be one or more layers of smooth muscle cells (8). Another important cell type in the vessel wall is the fibroblast. Fibroblasts are derived from mesenchymal cells and can be abundant in the outer walls of vessels, especially proximal arteries, where they form the so-called adventitial layer along with extracellular matrix. The relative number of cells and thickness of the smooth muscle (medial layer; and fibroblasts (adventitial layer) varies considerably between proximal and distal vessels. At the level of the capillaries, most studies suggest that pericytes are the primary support cells.
Recently a study by Peng et al. (5) examined the origins and development of the pulmonary arteries and veins that connect the lung to the heart (Figure 3-7). They examined development of these major vessels in a lung agenesis model created by deletion of β-catenin from the anterior foregut (AFG) endoderm. They showed that the pulmonary arteries and veins still develop in the absence of lung development and intersect in the region where the lung normally forms. In β-catenin knockout mice, the main pulmonary arteries and veins persisted through embryonic development, although they did not branch or develop further. What is especially important about this study is that Peng and colleagues used lineage tracing and conditional gene deletion experiments to identify a common population of cardiopulmonary progenitor (CPP) cells that give rise to the mesenchymal cells of the cardiac inflow tract and the lung, including proximal endothelial cells, vascular smooth muscle cells, and pericyte-like cells as well as airway smooth muscle (Figure 3-7). These findings are consistent with suggestions by Hall et al. (9) that airway and vascular smooth muscle cells have a common origin. Peng et al. (5) further suggested that endothelial cells in the distal capillaries (in the alveolar regions) are not derived from CPP cells, but from a different population of mesenchymal progenitor cells that express VE-cadherin. When it comes to alveolar development, there is ample evidence that it is driven by the architecture of the capillaries as reviewed in (10).
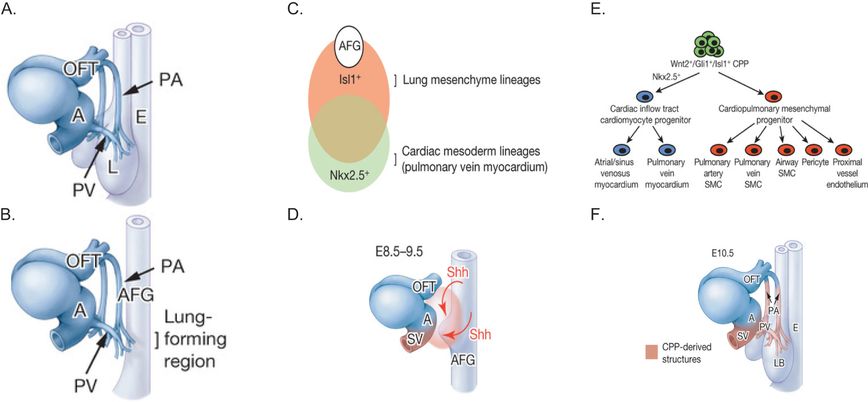
Panel A: The schematic shows the large pulmonary arteries (PA) and pulmonary veins (PV) in relation to the developing esophagus (E), outflow tract (OFT), and atrium (AT) of the heart and the lung (L) at E10.5 in the mouse. Panel B: The PA and PV still develop in ShhCre Ctnnb1flox/flox mutants, despite the absence of lung specification. Panel C: Schematic depicts lung and cardiac mesodermal lineages, overlap, and molecular markers (Isl1 and Nkx2.5, respectively) in relation to the anterior foregut (AFG). Panel D: Schematic shows how sonic hedgehog (Shh) produced by the AFG endoderm stimulates cardiopulmonary mesoderm progenitor cells (orange zone) to form the pulmonary artery (PA) and pulmonary veins (PV). Panel E: The schematic shows how the different lung mesenchymal cell populations develop from cardiopulmonary mesoderm progenitor (CPP) cells. Panel F: Schematic shows the vascular structures formed from cardiopulmonary progenitor (CPP) cells. LB, lung bud; E esophagus; A, atrium; OFT, outflow tract; SV, sinus venosus.
Cellular and Molecular Mechanisms
The developing airways are thought to play a major role in the regulation of pulmonary vascular morphogenesis. As mentioned earlier, pulmonary arteries closely follow the conducting airways, and the lung epithelium is a source of important paracrine factors, including sonic hedgehog and vascular endothelial growth factor (VEGF; see following), whose cognate receptors are located in the mesenchyme. In the following sections we will discuss factors that are known to regulate development of the pulmonary arterial system. Many more genes/factors have been identified as regulating vascular development in other organs or animal models that do not participate in lung development. In addition, mice in which many of the genes for these vascular factors have been inactivated die prior to or during the early stages of lung morphogenesis, and so the specific role that these factors play in pulmonary vascular development remains unclear.
Sonic Hedgehog and Patched: Sonic hedgehog (Shh) has a critical role in early patterning of the lung and branching morphogenesis. Shh is expressed at high levels in the distal tips of the epithelium. The receptor for Shh is patched (ptc), and it is expressed at high levels in the lung mesenchyme. Overexpression of Shh in the distal epithelium increased proliferation of mesenchymal and epithelial cells and resulted in an abundance of mesenchyme (11). These and other studies (12) show that Shh plays an important role in regulating mesenchymal and epithelial cell populations and patterning of the lung. However, whether Shh directly induces angioblasts and or endothelial cell differentiation or patterning of the vascular network in the lung mesenchyme is unclear. In the study by Peng et al. (5), an important role for Shh was identified in development of the main pulmonary arteries and veins that connect the heart to the lung. The vascular plexus was disorganized and also failed to connect to the heart in Shh–/– mice. Shh is expressed in the anterior foregut (AFG) adjacent to the cardiac inflow tract and at the time that pulmonary vascular development is first initiated. Gli is a downstream effector of hedgehog signaling and is expressed in CPP cells along with Wnt2 and Isl1. Inactivation of smoothened (Smo), which is important for Shh signaling in CPP cells, also disrupted pulmonary vascular plexus formation. Hence, this important study (5) suggests that Shh from the AFG signals to CPP cells to promote formation of the vascular plexus and also connections of the pulmonary arteries and veins to the outflow and inflow tracts of the heart.
Fibroblast Growth Factors: Fibroblast growth factors (FGFs) are important for induction of the primitive mesoderm in the early embryo. In Xenopus embryos, which lack FGF receptor-1 activity, vasculogenesis is inhibited, and endothelial cells are not induced (13). In the quail embryo, FGF-2 induces the formation of angioblasts, vascular patterning, and expression of the VEGFR2 receptor, Flk (14,15). FGF-2 may play an important role in the induction of angioblasts, which are subsequently responsive to VEGF. FGF-2 is expressed in epithelial and mesenchymal cells of lungs from midtrimester human fetuses (16). Although FGF-1, -2, -7, -9, -10, and -18 are expressed in the developing lung [reviewed in (17)], the role of these FGFs, and especially FGF2, in regulating angioblast induction in the splanchnic mesenchyme and pulmonary vascular development remains unclear. Cardiac mesoderm may be important for patterning the foregut endoderm from which the lung buds arise, potentially through FGF-1 and -2 (18). Whether cardiac mesoderm induces angioblasts in the splanchnic mesoderm via FGF2, and so initiates pulmonary vascular morphogenesis, is unclear.
Vascular Endothelial Growth Factors: Vascular endothelial growth factors (VEGF) are a family of factors that contribute to vessel development and function in a number of organs (19, 20). VEGF-A stimulates vasculogenesis and angiogenesis through its stimulatory effects on the differentiation and growth of endothelial cells. VEGF gene ablation in mice causes severe cardiovascular abnormalities and is embryonic lethal. Even heterozygous mice die at E10.5, with abnormal spatial organization of vessels and impaired differentiation of endothelial cells. Hence, even a moderate deficiency in VEGF may be detrimental to lung development and function. VEGF acts through two receptors, VEGFR1 (flt-1) and VEGFR2 (Flk or KDR in humans). The VEGFR2 is an early marker of angioblasts and is vital for both promitotic and prosurvival effects of VEGF on endothelial cells. VEGF plays an important role in the differentiation of angioblasts into endothelial cells (21). There are several isoforms of VEGF that are generated by alternative splicing from a single gene (22). Differential expression of these isoforms reflects distinct functions during vascular development (23). The larger isoforms, including VEGF 206, 188 and 164, bind to heparin proteoglycans and extracellular matrix and are not as freely diffusible as the smaller VEGF 120 isoform. VEGF 164 has intermediate properties as it can diffuse as well as bind to heparin proteoglycans (20). During the early pseudoglandular stage of lung morphogenesis in mice, VEGF expression is diffuse and present in both mesenchyme and epithelial compartments (24). As development proceeds, VEGF expression becomes increasingly restricted to the epithelium, and by E13-15 VEGF expression is most intense in the tips or leading edges of the branching airways, where it stimulates vascular morphogenesis in the surrounding mesenchyme (24).
In the saccular phase (E18 onward) and postnatally, VEGF expression becomes restricted to the distal lung epithelium and specifically alveolar type II cells (23,24). Analysis of the different isoforms of VEGF-A show that expression levels of VEGF 164 and 120 are relatively constant throughout pre- and postnatal phases of lung morphogenesis. VEGF 188, however, has low expression early and then increases from E13 through birth, remaining high after birth even into adulthood (23). The relative importance of the larger VEGF isoforms has been explored in mice in which VEGF 188 and 164 were deleted leaving only VEGF 120 (20,25). VEGF 120 deficient mice developed to term, but died at birth with abnormalities in pulmonary vascular development (23,25). The role of VEGF after birth was studied by Gerber and colleagues (26) by conditionally deleting the VEGF-A gene and treating newborn mice with a soluble VEGF receptor. In both cases, the mice failed to thrive, and morphogenesis in many organs, including the lung, was disrupted. Inhibition of VEGF receptor signaling in newborn rats also disrupted postnatal vascular and alveolar formation, underscoring the interdependency between vascular and airway development, at least in the final postnatal phase of lung development (27). It is apparent that strict control of VEGF levels in the lung is crucial for normal vascular development and function. Although loss-of-VEGF function is highly detrimental, prenatal overexpression of VEGF-A164 also disrupted lung morphogenesis (28,29), and postnatally elevated VEGF-A164 levels caused pulmonary vascular leak and alveolar hemorrhage (30). VEGF-D is also expressed in the developing lung and the distinctive patterns of VEGF-A and –D expression have been suggested to represent the unique functions that these different forms of VEGF play in lung development (31). VEGF-D and-C bind to VEGFR-3 and play an important role in lymphatic development.
Epidermal Growth Factor Receptor and Ligands: The epidermal growth factor (EGF) receptor (EGFR) and its ligands, transforming growth factor-α (TGF-α), EGF, heparin-binding EGF, and amphiregulin, contribute to epithelial development, maturation, and repair. There are conflicting reports on the roles of EGFR ligands in vascular development. In the hamster cheek pouch assay, TGF-α and EGF were found to be potent proangiogenic factors (32). However, in the avian embryo, TGF-α and EGF reduced the hemangiopoietic potential and endothelial cell migration from the splanchnopleural mesoderm (33). In the lung, overexpression of TGF-α and activation of EGFR signaling disrupt airway and vascular morphogenesis prenatally and postnatally (34–36). Lung development in TGF-α null mice appears normal, and interestingly, the mice are protected from the harmful effects of agents like bleomycin that induce pulmonary fibrosis (37). Knockout of the EGF and EGFR genes in mice is fetal lethal early in development, so their role in pulmonary vascular development remains elusive.
Angiopoietins and Tie Receptors: Tie1 and Tie2 (Tek) belong to a family of tyrosine kinase receptors that are specifically expressed by endothelial cells, including early in vascular development (38). The ligands for Tie receptors are the angiopoietins (Ang) and can have similar structures but also different and opposing effects on vascular development. Although angiopoietins can stimulate angiogenesis in vitro, most in vivo studies indicate that the angiopoietins and the Tie receptor system play more of a role in the secondary stages of vessel formation, that is, vessel assembly, remodeling, and maturation. The role of the angiopoietins and Tie receptors in lung development is unclear because gene deletion causes embryonic lethality at E10.5–12.5, during the early stages of lung development. Expression of Ang1 is altered in pulmonary diseases, including upregulation in patients with pulmonary hypertension (PH) and may play a role in the disease pathogenesis because increases in Ang1 in an animal model caused PH (39,40). Ang 1 is downregulated in infants with congenital diaphragmatic hernia (CDH), consistent with the pulmonary hypoplasia in these patients (41). However the role of the Ang/Tie system in pulmonary vascular morphogenesis remains uncertain.
Notch System: The Notch system regulates cell fate decisions in all three germ layers and plays important roles in neural, retinal, limb, heart, and vascular morphogenesis, as well as hematopoiesis (42). Four notch receptors have been identified, and ligands include Jagged 1–2 and Delta-like 1, 3, and 4. Deletion studies show that although the Notch system and its downstream signaling molecules are not necessary for vasculogenesis, they are essential for correct remodeling of the early vascular plexus. In mice loss of Notch 1 and 4, or Jagged 1 results in severely disorganized vasculature and is lethal at E9.5–10.5 (43, 44). Studies in zebrafish have shown that the Notch system is also involved in arterial–venous specification (45, 46). In early development the Notch receptors and ligands are expressed throughout the developing vascular system, but later expression becomes restricted to the arterial system. In zebrafish a hierarchical system has been determined in which Shh regulates VEGF-A and VEGF-A regulates Notch. Loss of gridlock, which is downstream of Notch signaling, caused loss of the arterial markers such as ephrinB2 and increased expression of the ephrin receptor EphB4, a venous marker. In mammals, arterial specification may be regulated by VEGF-A acting though Notch and ephrins and Hey1 and 2 that are downstream of Notch (47). Deletion of Hey 1 and 2 as well as Notch 1 in mice blocked vascular remodeling and caused loss of arterial markers, including ephrin B2 (48). Notch 3 plays an important role in arterial differentiation and maturation of vascular smooth muscle cells (49). Notch 1, 2, and 3 as well as Jagged-1 and-2 are expressed in the developing lung (50). Notch-1 and Jagged-1 and -2 are expressed in both the epithelial and mesenchymal compartments at E14. Although inhibition of Notch-1 expression in fetal lung explant cultures, but not -2 or -3, increased epithelial branching, effects on vascular morphogenesis were not reported. In the lung Notch-2 is regulated by one of the Foxhead box (Fox) transcription factors, Foxf1 (51). The role of Notch signaling in pulmonary vascular morphogenesis remains poorly understood.
Transforming Growth Factor-β, endoglin, Activin Receptor Kinase, and Bone Morphogenic Proteins (BMPs): The transforming growth factor-β (TGF-β) superfamily includes the three isoforms of TGF-β, activins, inhibins, growth and differentiation factors as well as the bone morphogenic proteins (BMPs). Heteromeric complexes of two types of serine/threonine transmembrane kinase receptors bind TGF-β superfamily members and activate downstream Smad proteins. TGF-β plays a vital role in vasculogenesis and angiogenesis, as well as differentiation and maturation of new vessels (52, 53). Activated TGF-β is released by endothelial cells on contact with pericytes. TGF-β inhibits endothelial proliferation and stimulates recruitment and differentiation of mural cells, such as pericytes and smooth muscle cells. TGF-β also stimulates deposition of extracellular matrix that is critical for the structure of larger vessels. Endoglin is an auxiliary receptor that interacts with type II receptors for TGF-β and activin. Loss of endoglin in mice causes a similar phenotype to loss of TGF-β, suggesting that endoglin is necessary for TGF-β signaling during vascular development (54). Endoglin expression is seen very early in endothelial cells throughout the developing embryo and is strongly expressed in lung mesenchyme (55). Expression of endoglin and Alk1 are similar, and Alk-1, one of the seven type I TGF-β receptors, is expressed by endothelial cells and is critical for the effects of TGF-β on vascular development (56). Alk-1 null mice die in midgestation from vascular defects characterized by excessive fusion of the capillary plexus into cavernous vessels, hyperdilation of larger vessels, and defective recruitment and differentiation of smooth muscle cells (56,57). The role of TGF-β in vascular development may be more complex than was initially appreciated. In proximal tubular cells from the kidney, TGF-β regulates expression of VEGF-A, and angiostatic factors, thromospondin-1 (TSP-1), and the soluble VEGF receptor, sFlt-1 (58).
BMPs play important roles in vascular development, and BMP receptors are expressed by endothelial and smooth muscle cells. BMP-2/4, BMP-5/7, and Smad1 and-5 deficient mice have enlarged vessels and reduced numbers of smooth muscle cells (59). BMP6 is expressed by endothelial cells and smooth muscle cells and stimulates endothelial cell migration and tube formation through induction of Id1 a helix-loop-helix transcription factor that can repress expression of thrombopsondin-1 (TSP-1) that is a potent inhibitor of angiogenesis (60). In the lung most studies have focused on BMP4, as it plays an important role in epithelial–mesenchymal interactions [reviewed in (17)]. Early expression of BMP4 is in the splanchnic mesoderm near the location where the lung buds arise from the foregut endoderm, and then later BMP4 expression is in the proximal mesenchyme and distal epithelial tips. However, whether BMP4 directly regulates pulmonary vascular morphogenesis is unclear. Mutations in the BMPRII receptor gene have been linked to pulmonary vascular diseases such as familial pulmonary arterial hypertension (FPAH), principally seen in adults (61). Interactions between BMPs and the Notch system have been reported, in that BMPs blocked the inhibitory effects of Notch signaling on endothelial cell migration (62). BMPs signal in pulmonary arterial endothelial cells and smooth muscle cells by activating downstream effectors of the Wnt signaling pathway. In endothelial cells (63), BMPs activate β-catenin and PPARγ to form a complex that regulates downstream genes in endothelial cells such as apelin that are important in angiogenesis (64). In smooth muscle cells, BMPs transiently activate β-catenin to regulate downstream genes that influence migration (65).
Wnts: Wnts are secreted growth factors that regulate important cell processes, including proliferation, polarity, differentiation, and angiogenesis (66). The Wnt family comprise 18 or more ligands that signal through 10 known frizzled receptors (Fz). Wnt-ligand binding activates multiple intracellular pathways including the β-catenin/LEF-TCF pathway. Wnt-5a, -7a, and -10b are expressed by endothelial cells in vitro, and Wnt-5a by vascular smooth muscle cells (67). Wnt-2 is important for placental vascular development, as fetal-derived placental capillaries were reduced in Wnt-2 –/– mice, resulting in high perinatal mortality and growth restriction of surviving pups (68). The role of Wnts in pulmonary vascular morphogenesis is not well understood. However, Wnt7b is expressed at high levels in the epithelium of distal airways, and inactivation of the Wnt7b gene in mice resulted in perinatal death likely from the lung hypoplasia and pulmonary hemorrhage seen in these animals (69). Examination of lung histology showed that the smooth muscle of the large vessels was hypertrophic and apoptotic, which likely accounted for the vessel rupture and pulmonary hemorrhage. Wnt7b is required for expression of extracellular matrix protein tenascin-C (TN-C) that it in turn can regulate pdgfr-α and pdgfr-β expression and proliferation of smooth muscle precursor cells (70).
Platelet Derived Growth Factors: PDGF-A and –B are involved in vessel remodeling and maturation; however, whether PDGF-A regulates angiogenesis is unclear (71, 72). PDGF-B and its receptor PDFG-β can be simultaneously expressed by endothelial cells, suggesting an autocrine role in angiogenesis. Endothelial cells in large vessels express PDGF-B but not the PDGF-β receptor, suggesting a paracrine role, but capillary endothelial cells respond to PDGF-B by increasing capillary density. Interestingly, one study showed that VEGF-A induces VEGFR2-positive embryonic stem cells to differentiate into endothelial cells, whereas PDGF-BB caused VEGFR2-positive embryonic stem cells to differentiate into pericytes and vascular smooth muscle cells (73). This study addresses an important concept, namely that different growth factors can stimulate the differentiation of potentially a common pool of vascular progenitor cells into different lineages that will form endothelial cells and mural support cells like smooth muscle cells, fibroblasts, and pericytes. Hence, PDGFs are likely important in recruitment and differentiation of mural cells that are critical for vascular structure and function. Whether PDGFs play a similar role in stimulating initial development of vessels in the lungs is unclear, although there are studies examining the role of PDGFs in pulmonary vascular diseases, such as PAH (74).
Insulin-like Growth Factors: The insulin-like growth factor (IGF) system is a complex system that includes two ligands (IGF-1 and IGF-2), two receptors (IGFR1 and IGFR2), at least six IGF binding proteins (IGFBP-1 to-6) and proteases that regulate IGF activity (75). IGF signaling regulates cell proliferation and differentiation and whether cells undergo mitosis or apoptosis. IGF signaling can regulate embryonic development as well as postnatal growth, body size, and longevity. IGFs play an important role in development of a number of organs (75). IGF-1 stimulates proliferation of endothelial cells in vitro, and IGF-1/II can stimulate angiogenesis in the retina. Expression of IGF-I and IGF-II and IGFR-1 receptor have been reported in airway epithelial cells and smooth muscle cells adjacent to the airways, as well as endothelial cells lining the primary vascular plexus of 4- to 12-week-old human lung tissue (76). Treatment of human fetal lung explants with an IGFR-1 blocking antibody increased apoptosis in mesenchymal cells, particularly endothelial cells, and disrupted epithelial branching. Treatment of fetal rat lung explants with the IGFR-1 antibody caused loss of blood islands in the mesenchyme. Hence, there is evidence to support a role for IGFs, acting through IGFR-1, in pulmonary vascular morphogenesis.
Ephrins: Ephrins and Eph receptors are widely expressed throughout the developing embryo and play important roles in vascular development (77). In the ephrin/Eph receptor system, both ligand and receptor must be membrane bound, so that ephrin ligands can cluster and activate receptor signaling. As a result ephrin/Eph signaling interactions are mostly restricted to neighboring cells and important in local cell-to-cell interactions rather than long-range signaling. Ephrin-B1 and -B2 can promote capillary-like assembly of cultured endothelial cells and angiogenic sprouting. Genetic ablation of ephrin-B2 in embryonic mice resulted in failure of the primary capillary network to remodel and so disrupted vascular development (78). The ephrin/Eph system likely has a role in arterial versus venous specification because ephrin-B2 is expressed in embryonic arteries but not veins, whereas its receptor, EphB4, is expressed in veins but not arteries (79). However, ephrin-B1 is expressed in arterial and venous endothelial cells and EphB3 in veins and some arteries. Ephrin-B2 expression persists in adult arteries and also extends into microvessels, raising into question the concept that capillaries have neither arterial nor venous identity (80). Ephrin-B2 and EphB2 are expressed by mesenchymal cells adjacent to endothelial cells, suggesting that ephrins might play a role in endothelial-mesenchymal interactions (79). Ephrin-B2 is also expressed in the smooth muscle of arteries as well as endothelial cells, but not in venous smooth muscle and indicates a major difference between these smooth muscle beds (80). Expression of ephrin-B2 in arterial smooth muscle cells after endothelial cells suggests that a signal from arterial endothelial cells may be inducing expression of ephrin-B2 in vascular smooth muscle cells. Ephrin-A1, -B1, and -B2 and EphB4 are expressed in capillary endothelial cells from adult mouse lungs, but the role of ephrin/Eph in pulmonary vascular morphogenesis remains unclear.
Angiostatic Factors: A number of naturally produced factors that can inhibit angiogenesis have been identified (81), including angiostatin, endostatin, TSP-1, pigment epithelial derived factor (PEDF), soluble Flt-1, endothelial-monocyte activating polypeptide (EMAPII), and fibulin-5. Angiostatin and endostatin are not produced as angiostatic factors de novo, but are proteolytic fragments of plasminogen and type XVIII collagen. In contrast, TSP-1, and PEDF are produced directly from distinct genes, and hence their angiostatic activity appears to be one of their primary functions. Although there is evidence that these factors regulate vascular morphogenesis in organs, most attention has been focused on the role of these factors in pathologic processes, such as tumor vascularization and their potential use as antitumor agents (82). Therefore while angiostatic factors are expressed in the lung and altered in disease states, such as lung cancer, pulmonary fibrosis, acute respiratory distress syndrome (ARDS), and emphysema, the role of these factors in pulmonary vascular morphogenesis is not well understood. While lung development appears normal in TSP-1 null mice, there is massive inflammatory cell influx into the lungs after birth, suggesting a role for TSP-1 in lung homeostasis (83), which may be due to the role of TSP-1 in regulating TGF-β (84). PEDF may exert its angiostatic effects by downregulating VEGF expression, although its role in pulmonary vascular morphogenesis is unclear. Alternative splicing of the VEGFR1 or Flt-1 gene generates a soluble form of the receptor, sFlt-1, which is a potent natural antagonist of VEGF. sFlt is expressed in the lung, although its role in normal pulmonary vascular development is also unclear. However, one study showed that excess sFlt-1 in amniotic fluid during late gestation reduced alveolarization and pulmonary vascular growth in infant rats, suggesting that it may play a functional role (85). EMAP II is a cytokine-like molecule that has potent angiostatic activity, inducing cultured endothelial cells to undergo apoptosis. Expression of EMAPII was high during the pseudoglandular stage of lung morphogenesis, but decreased as pulmonary vascular morphogenesis progressed (86). EMAPII may be a negative regulator of pulmonary vascular growth based on fetal lung allograft studies. Hence, there may be interactions between angiostatic factors and proangiogenic factors, which may represent regulatory or homeostatic feedback loops. The presence of such feedback loops would not be surprising given the need to carefully regulate vascular morphogenesis in proportion to airway development.
MicroRNAs: MicroRNAs can regulate networks of genes by binding to multiple RNAs, generally to inactivate and degrade them, thereby transforming cellular phenotype. Micro RNAs are important in angiogenesis and vascular development of the lung, miR-221 being antiangiogenic and miR-130 being proangiogenic (87).
Transcription Factors: A number of transcription factors regulate endothelial cell specification and differentiation into arterial, venous, and lymphatic lineages. Some examples are discussed in the following paragraphs, including the hypoxia-inducible factors (HIF), Hox genes, Fox genes, Sox genes, and members of the GATA family. The reader is directed to reviews for more information (88–90). Our understanding of the role of these factors in vascular development comes from studies in zebrafish and other models and a few studies in the developing lung.
Hypoxia-inducible transcription factors (HIFs) have been extensively studied because of their role in hypoxia responses. HIF-1α plays an important role in oxygen homeostasis and regulates VEGF (91,92). Deletion of the HIF-2α gene (also known as EPAS1) was recently shown to cause respiratory distress and death at birth in mice (93). HIF-2α null mice had reduced VEGF levels, but structurally the lungs of HIF-2α null mice did not appear grossly abnormal, except for an increased abundance of immature pneumocytes and some subtle deficits in lung vascularization later in gestation.
Hox transcription factors are mammalian homologues of homeobox genes that regulate body patterning and organogenesis in Drosophila. While deletion of some Hox genes disrupt lung morphogenesis, the role of these transcription factors in pulmonary vascular morphogenesis is not well understood (94). For example, Hoxb-5 regulates airway patterning, possibly by its effects on tenascin-C and FGF10 expression, and so may indirectly affect vascular development (95). Paired-related homeobox gene (Prx1) has been implicated more directly in pulmonary vascular development. Prx1 is expressed in endothelial cells and the wall of muscularized vessels early in lung morphogenesis and then later in the medial and adventitial layers (96). Ihida-Stansbury and colleagues suggested that Prx1 plays a role in promoting patterning and differentiation of vessels in the developing lung based on in vitro studies in which Prx1 transfection into fetal lung mesenchymal cells induced an endothelial-like phenotype and the ability to form vascular networks in matrigel (96). Furthermore, Prx1–/– mice were cyanotic, die soon after birth, and had decreased expression of TN-C, which may have contributed to the hypoplastic lung phenotype and lack of pulmonary vessels in these animals.
Fork-head box (Fox) transcription factors are expressed in mesoderm, including the splanchnic mesoderm, and are important in mesenchymal induction of the lung epithelium. Haploinsufficiency of Foxf1 causes abnormal development of the lung, as well as the gall bladder, esophagus, and trachea. Lung abnormalities in Foxf1+/– mice included hemorrhage, fusion of lung lobes, and defects in saccule and microvascular development (97). Reduced expression of Notch2 and Hes-1, its downstream target, may contribute to the abnormalities caused by Foxf1 haploinsufficiency (51). FoxC1 and FoxC2 have virtually identical DNA-binding domains. Mice that were double mutants for FoxC1 and C2 had arteriovenous malformations, and lymphatic endothelial cells failed to sprout off from the veins likely due to reduced VEGF-C (98). While expression of the venous marker COUP-TFII was normal, double-mutant mice lacked expression of arterial markers, suggesting that FoxC transcription factors play an important role in arterial cell fate specification. However, the role of FoxC1 and C2 in the lung is unclear.
SRY-related high-mobility group box (Sox) transcription factors comprise a family of 20 different members. Studies suggest that the Sox F group, which includes Sox 7, 17, and 18, regulate arteriovenous specification and venous lymphatic specification. Loss of one Sox F member may be compensated for by the other F group members (90). Sox18 has raised a lot of interest, as it can regulate Prox1 and therefore lymphatic development. Sox17 is expressed in mesenchymal progenitors of endothelial cells in the developing lung and is solely found in endothelial cells in the adult lung (99). Conditional deletion of Sox17 in mesoderm resulted in abnormal pulmonary vascular development, including enlarged arteries, vein varices, and decreased microvasculature with alveolar hypoplasia.
There are six GATA transcription factors, of which GATA-1, -2, and -3 play important roles in hematopoietic cells. Studies suggest that GATA-2 regulates differentiation of endothelial cells (90) and key endothelial genes, including VEGFR2 (Flk-1), endomucin, and VEcadherin (100). Knockdown of GATA2 in dermal endothelial cells reduced expression of these genes and increased nonendothelial genes “including” smooth muscle actin and SNAIL, suggesting that failure to progress into an endothelial cell fate leads to direction into other mesenchymal cell fates like smooth muscle.
Extracellular Matrix Molecules: Extracellular matrix (ECM) molecules provide important structural integrity to mature vessels to support their function and a scaffold and cell adhesion that supports the growth and development of vessels. Growth factors such as VEGF, especially the longer isoforms, bind to ECM, although the exact role of this function is not entirely clear. ECM proteins include collagen, laminin, fibronectin, elastin, and tenascin. Collagen and lamin are produced early in lung development and increase as development proceeds. In contrast, fibronectin expression increases in the pseudoglandular stage when preacinar arteries are forming and then decreases during the canalicular phase (101). Elastin levels also increase in the pseudoglandular stage and continue to rise in the saccular and alveolar stages, when it plays an important role in alveolar and vascular development and structure (102). In transgenic mice, even haploinsufficiency of elastin results in pulmonary hypertension later in life (103).
Integrins: Integrins are composed of α and β subunits that form transmembrane heterodimer complexes. Integrins function as cell surface receptors that interact with other proteins, including ECM. The β1 integrin family includes receptors that recognize collagen, laminin, and fibronectin. The β3 integrins include receptors for fibronectin, von Willebrand factor, and thrombospondin. Integrin expression by endothelial cells is complex [see review by Albelda (104)]. The five integrins that contain the αv subunit are widely expressed in most tissues in mice. However, mice that lack the αv subunit, and are therefore deficient in all five integrins, develop normally, suggesting that lung development and cellular differentiation can occur in the absence of these integrins (105).
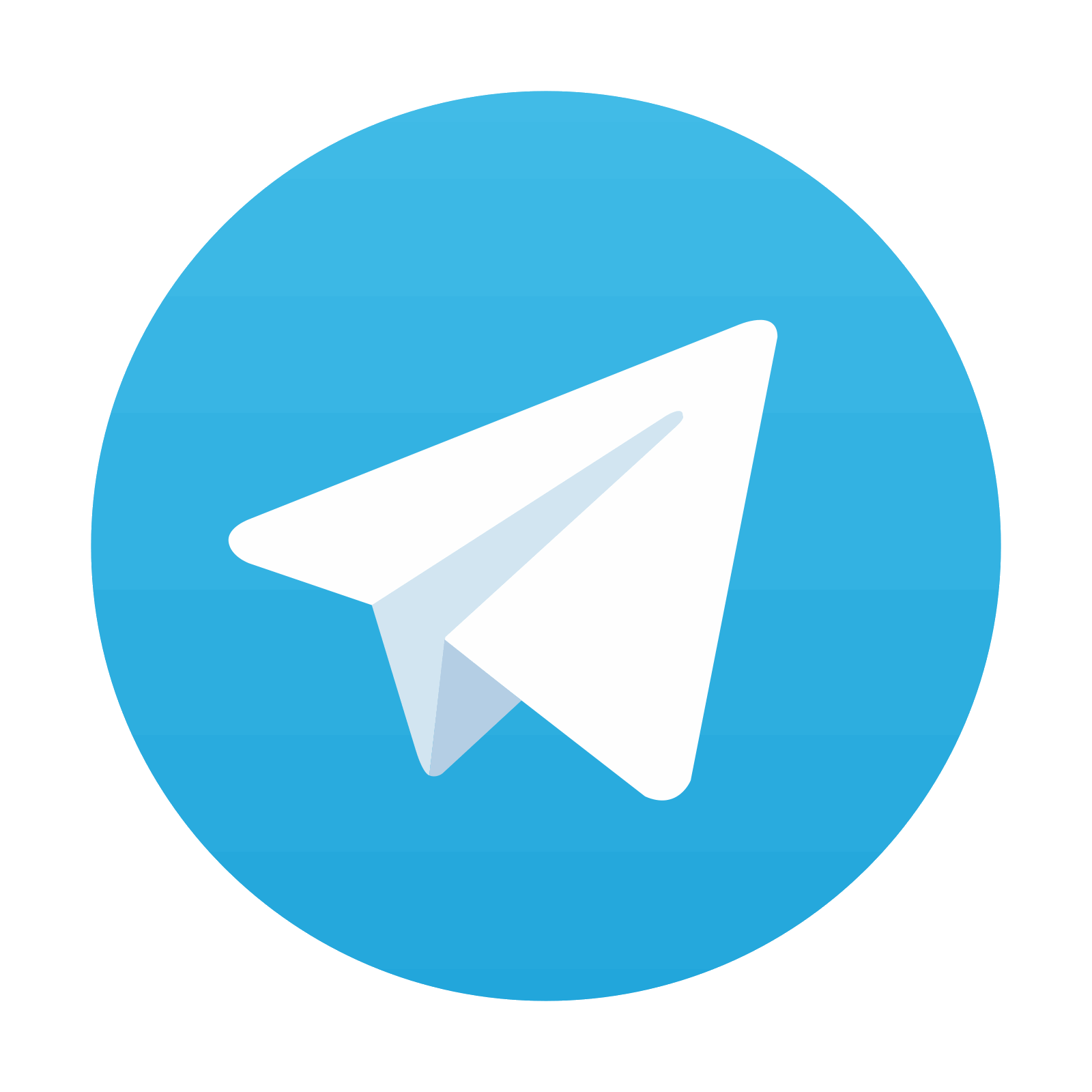
Stay updated, free articles. Join our Telegram channel
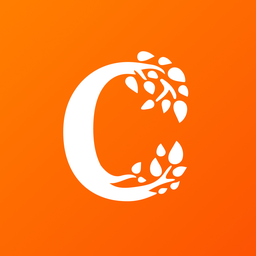
Full access? Get Clinical Tree
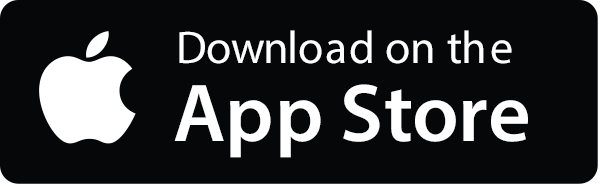
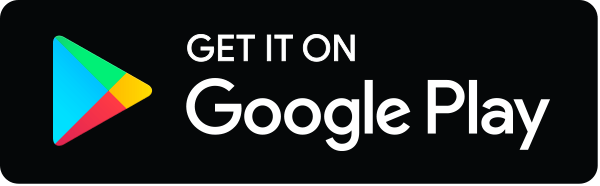