Pulmonary hypertension (PH) itself is not a disease and does not specify any unique pathophysiology. PH refers to elevated pulmonary artery (PA) pressure.
The underlying physiology is variable with a major distinction between PH caused by elevated pulmonary venous (distal) pressure, elevated pulmonary vascular resistance (PVR), and high flow (Figure 9-1).1
Pulmonary hypertension can be due to any combination of these 3 underlying physiologies in various types of congenital heart disease. Marked pulmonary vascular remodeling does not necessarily translate into elevated pulmonary artery pressure in the setting of low flow. The presence of dynamic shunting complicates interpretation. An acute increase in flow itself causes recruitment and dilation of pulmonary vessels, resulting in decreased pulmonary vascular resistance.
While the equation for PVR (PVR = ΔP/CO) may suggest that PVR is equivalent at any flow (eg, if you double CO then ΔP will double and therefore PVR will remain stable), the relationship is not linear (Figure 9-2). While this phenomenon can be important in the absence of congenital heart disease (CHD), its appreciation is critical in interpretation of hemodynamic data in patients with shunting.
Further, distinct underlying etiologies often produce equivalent physiology and indistinguishable pulmonary vascular pathology.
Pulmonary arterial hypertension (PAH) is defined as PH with high PVR without pulmonary venous hypertension.2
This chapter will review PH in adults with congenital heart disease from the perspective of the underlying physiology followed by several cases which highlight dynamic interplay between the component parts of “pulmonary pressure” and finally a discussion of special cases of right heart pathology requiring special consideration.
FIGURE 9-1
The hemodynamic underpinnings of elevated pulmonary artery pressure. Elevated left-sided filling (or distal) pressure is the most common reason for elevated pulmonary artery pressure in the general population, and is a contributor in a significant subset of patients with congenital heart disease. High pulmonary vascular resistance is the hemodynamic cause of elevated pulmonary pressure in pulmonary arterial hypertension (PAH) of various causes. Increased pulmonary flow is seen in patients with left-to-right shunting, and defining the extent of flow is critical in the assessment of shunt lesions. There is important overlap and the findings are flow-dependent and may be dynamic for a given patient depending on the context. That is, distal pressure and pulmonary vascular resistance may vary at different levels of pulmonary flow.

FIGURE 9-2
The relationship between mean pulmonary artery pressure and mean flow (ie, pulmonary vascular resistance) is not equivalent at all levels of flow as a result of vessel recruitment and distention with higher flow. Therefore, resistance (the ratio of pressure to flow) is lower at higher flow. PAP, pulmonary artery pressure.

There are a number of approaches to determine the physiologic underpinning of elevated pulmonary artery pressure in a given patient with congenital heart disease.
Complete understanding of the underlying defect and prior procedures is essential and this information alone usually provides a good sense of the most likely reason a patient has high PA pressure.
However, many defects may be associated with high flow, high resistance, or high distal pressure. Atrial septal defect (ASD) is a common example where this distinction is critical and this chapter will review several cases of patients presenting with ASD and pulmonary hypertension, each of which constitutes a different clinical picture.
Physical examination is helpful in exploring the cause of high PA pressure, as is the 12-lead electrocardiogram and chest radiograph. In the current era, however, it is rare that a patient does not undergo more technologically involved cardiac imaging and, sometimes, catheterization.
While pulmonary function testing, cardiac magnetic resonance imaging (MRI), cardiac computed tomography (CT), and nuclear imaging have a role in evaluation in a subset of patients, the vast majority of clinically actionable data on pulmonary hypertension in adult congenital heart disease (ACHD) is derived from echocardiography and catheterization.
These 2 common techniques will be reviewed in further depth.
An isolated unexpected finding of elevated PA systolic pressure on a transthoracic echocardiography is a common reason for the initial suspicion that a patient has PH.
The PA systolic pressure is estimated using the modified simplified Bernoulli equation (ΔP = 4V2) from the systolic transtricuspid velocity adding a value for estimated right atrial (RA) pressure (whether a constant based on examination or the size and pattern of inferior vena cava [IVC] collapse) (Figure 9-3). This is the extent of the evaluation for many patients. This is unfortunate not only because pulmonary artery systolic pressure (PASP) is only moderately accurate by echocardiography,3 but even more importantly because even when accurate, the PA pressure provides no information on why there is elevated pulmonary artery pressure.
Also unfortunate, adequate tricuspid regurgitant jets are not present in all patients and the echocardiographic evaluation often does not extend beyond this single measurement.
On a more positive note, there are several very simple variables that can be gleaned from clinically obtained echocardiograms that provide further insight.
In addition to PA systolic pressure, PA diastolic and mean pressures can also be estimated using echocardiography. The most common, though not the only, method to estimate diastolic pressure uses the estimated gradient between main PA and right ventricular (RV) outflow tract by measuring transpulmonic valve end regurgitant velocity (ΔP ≈ 4 × V2). This value added to the estimated right atrial pressure approximates end-diastolic PA pressure. Mean PA pressure can be estimated using the equation in Table 9-1.
Right ventricular geometry is complex, and RV volume and function are difficult to assess precisely with 2-dimensional echocardiography. Nevertheless, there are several simple parameters that provide relatively robust estimates of RV function in patients without for sake of uniformity-should congenital heart disease be CHD through the chapter (after the first time its used), though these must be used with caution in CHD patients. Tricuspid annular plane systolic excursion (TAPSE) describes the distance traveled by the tricuspid annulus toward the probe during systole using M-mode or 2D imaging. A low value (eg, <1.8 cm) argues for right ventricular dysfunction.
In patients with CHD with the real potential to have unappreciated RV dyssynchrony (eg, those with repaired tetralogy of Fallot who have had a prior right ventriculotomy and/or transannular patch placement), any single variable is likely to be misleading.
Late systolic flattening of the ventricular septum (quantified by the eccentricity index) is often reported as “RV pressure overload.”4 This is an excellent marker of elevated impedance to RV ejection, but may give a false impression that peak systolic RV pressure must be approximating left ventricular (LV) pressure to be able to “push the septum back” toward the LV. In fact, late systolic flattening is a relatively early sign of elevated pulmonary vascular impedance and is related to delayed and prolonged RV systolic time compared with the left ventricle.5, 6
There are also echocardiographic signs indicated adverse ventricular interaction in patients with a volume-loaded right ventricle, such as with atrial septal defect, but with normal pulmonary vascular resistance.7
There are more complex quantitative methods to estimate RV size or function such as fractional area change, but these are little used in clinical practice. The advent of 3-dimensional imaging promises improvements in echocardiographic RV quantification.
It is equally important to assess for evidence of elevated left atrial (LA) pressure or characteristics that predispose to left atrial pressure.8 Left atrial size, left ventricular hypertrophy, and abnormal diastolic indices are the most common. The presence of a dilated left atrium strongly suggests long-standing left atrial hypertension.
Note that “grade 1” diastolic dysfunction (reversed E:A) is often present in pulmonary arterial hypertension because of ventricular interaction and actually argues quite strongly against elevated LA pressure being the cause of elevated PA pressure. Tissue Doppler imaging provides important information on left atrial pressure, but lateral mitral annular velocities should be used since septal velocities are affected by right ventricular dysfunction or electrical delay, which are common in congenital heart disease.9
Pulsed-wave Doppler flow velocity of the right ventricular outflow tract is an excellent indicator of the pulmonary impedance. Several variables may be measured including acceleration time, velocity-time integral (VTI), and qualitative notching. Shorter acceleration time (the time from the initiation of flow to the peak velocity) correlates with higher pulmonary artery pressure and PVR (Figure 9-4).
Mid-systolic notching of the flow velocity envelope represents systolic flow deceleration in the setting of reflected pressure waves and strongly suggests elevated PVR and increased pulmonary artery stiffness (Figure 9-5).10 Notching is the Doppler correlate of the historically reported finding of a “flying W” pattern of pulmonary valve motion on M-mode imaging (Figure 9-6).
RAP = right atrial pressure (mm Hg) |
MAP = mean systemic arterial pressure (mm Hg) |
DPAP = diastolic pulmonary artery pressure (mm Hg) |
SPAP = systolic pulmonary artery pressure (mm Hg) |
MPAP = mean pulmonary artery pressure (mm Hg) |
PAWP = pulmonary artery wedge pressure (PA occlusion pressure and PA capillary wedge pressure are clinically equivalent terms) (mm Hg) |
HR = heart rate (bpm) |
CO = Qs = cardiac output (l/min) |
SV = stroke volume (mL/beat) = (CO × 1000)/HR |
BSA = body surface area (m2) |
CI = Qsi = cardiac index (l/min/m2) = CO/BSA |
SVI = stroke volume index (mL/beat/m2) = SV/BSA |
PVR = pulmonary vascular resistance (WU/m2 or dyne × second × s-5/m2) |
PVRI = pulmonary vascular resistance indexed to BSA = PVR × BSA) |
SVR = systemic vascular resistance (either WU or dyne × second × s-5) |
WU = Wood unit (mm Hg/l/min) |
1 WU = 80 dynes × seconds × cm–5 |
CaO2 = oxygen content of blood (mL/L) |
PaO2 = partial pressure of oxygen |
VO2 = O2 consumption (mL/min/m2) |
MPAP ≈ 0.67 × DPAP + 0.33 × SPAP |
Transpulmonary gradient (TPG) = MPAP – PAWP |
cO2 (mL/L) = (1.34 × [Hg] [g/dL] × SO2 × x 10) + (PaO2 × 0.003) |
cO2 (mL/L) ≈ (1.34 × [Hg] [g/dL] × SO2 × x 10) |
CO (Fick) = (VO2)/(systemic arterial cO2 – mixed venous cO2) |
PVR = TPG/CO |
SVR = (MAP – RAP)/CO |
CI = CO/BSA |
PVRI = TPG/CI |
SVRI = (MAP – RAP)/CI |
FIGURE 9-6
Pulsed-wave Doppler of the right ventricular outflow tract (RVOT) (top) and M-mode of the pulmonary valve (bottom) in a patient with pulmonary arterial hypertension. Red arrow identifies mid-systolic flow deceleration while the blue arrow identifies transient movement of the pulmonary valve toward the closed position (“flying W”) as a consequence, both related to reflected waves in the pulmonary circulation.

All patients with suspected pulmonary arterial hypertension (ie, high PVR) in the setting of CHD, without unusual extenuating issues, should undergo diagnostic catheterization.
The vast majority of pulmonary hypertension (ie, elevated mean PA pressure) in the general population is due to left heart disease and this is also true for a sizable subset of adults with CHD.
Discussion of the mechanics of catheterization and catheterization techniques in the presence of intracardiac shunting or complex defects is beyond the scope of this chapter, but it is important to review the fundamental hemodynamic evaluation of elevated PA pressure.
As such, a standard right heart catheterization (RHC) will be discussed.
For adults, right heart catheterization is usually performed with a single or double-lumen balloon-tipped radiopaque catheter with distance markings every 10 cm, with or without a near-the-catheter tip thermistor (for thermodilution output measurements). When 2 fluid-filled lumens are present the proximal lumen opens approximately 30 cm from the catheter tip and the distal lumen opens at the tip beyond the balloon. A third lumen is used to transmit gas or fluid from a syringe to the balloon, which usually has a capacity of 1.5 mL.
Intracardiac pressures should be measured with neutral intrathoracic pressure, most closely approximated at end expiration for spontaneously breathing patients. Mean pressures should generally not be substituted for end-expiratory pressure.
In the presence of marked shifts in intrathoracic pressure with breathing, often seen in patients with labored breathing, digitally averaged pressure over the entirely respiratory cycle may variably over- or underestimate true chamber; steps should be taken to limit respiratory variation such as encouraging an end-expiratory maneuver. The ability of patients to comply with such requests is critical, and support performance of catheterization with limited, if any, sedation to the extent possible.
Intrathoracic pressure can be estimated using esophageal manometry, but this is very rarely employed in clinical practice and has limitations.
It is important to confirm a PA “wedge” pressure. It can be difficult to completely obstruct antegrade flow in patients with PH; CHD patient with systemic-pulmonary arterial collateral flow also poses challenges to optimal wedge positioning.
With care not to dissect the occluded branch vessel or to extravasate, a small amount of contrast (see discussion of wedge angiography later) may be slowly injected to similarly confirm wedge position.
There are 2 methods to estimate cardiac output commonly used in clinical practice, indicator dilution and Fick.
Indicator dilution involves injecting an indicator, usually room temperature or cold saline, through a proximal catheter port. In the case of thermodilation there is a thermistor near the distal end of the catheter which measures temperature change after injection of a specified volume of saline of known temperature.
Using these data, cardiac output is estimated using the Stewart-Hamilton equation. Dilution techniques are inaccurate in the setting of intracardiac shunts, and the accuracy is questionable with severe tricuspid regurgitation or cardiogenic shock.
Measurement should be repeated at least 3 times (or more if measurements are not consistent) to ensure an accurate estimate. Dilution techniques do not require an estimate of oxygen consumption, which avoids an important source of error in the use of the Fick principle when this variable is assumed based on population data.
The quantity of O2 extracted from the blood is equivalent, during aerobic metabolism, to the amount of O2 used by the tissues. Whatever volume of blood the heart is able to deliver (cardiac output), the tissues must extract some absolute amount of oxygen to support a given level of aerobic metabolism.
If the cardiac output doubles, half as much oxygen per unit of blood will need to be extracted to support a given degree of oxygen consumption.
The Fick equation uses this relationship to estimate the cardiac output using oxygen consumption (measured or assumed/estimated) and the arteriovenous difference in oxygen content.
Cardiac output (l/min) = [oxygen consumption (mL/min)]/(arterial O2 content –venous O2 content). See Table 9-1.
The blood’s oxygen content is mostly composed of oxygen molecules bound to hemoglobin, with a small contribution by oxygen molecules in solution.
Catheterization should be performed with the patient using their baseline supplemental oxygen, and there is a negligible absolute amount of dissolved oxygen. Therefore, the estimate of oxygen content usually ignores dissolved oxygen. Only when a patient is on high concentrations of supplemental oxygen with high Pao2 does this importantly impact cardiac output estimates (see Table 9-1).
Oxygen consumption can be directly measured during catheterization. In clinical practice, however, O2 consumption is usually estimated using normative equations derived from population averages.
Many clinicians use a standard value of 125 mL/min/m2, though empiric normative values by age, sex, body size, and heart rate are available. At the extremes of body size and age, these estimates are of questionable validity even on a population scale.
On an individual basis, the inaccuracy of assumed vo2 constitutes one of the most important limitations of the accuracy of Fick of cardiac output estimates.11, 12, 13
PVR usually refers to the prevenous component of resistance. Resistance describes the relationship between pressure and flow, in this case between mean pulmonary artery pressure (MPAP) and pulmonary blood flow which approximates systemic cardiac output in the absence of intracardiac shunting.
The main hemodynamic parameters of interest in the evaluation of PH are as follows:
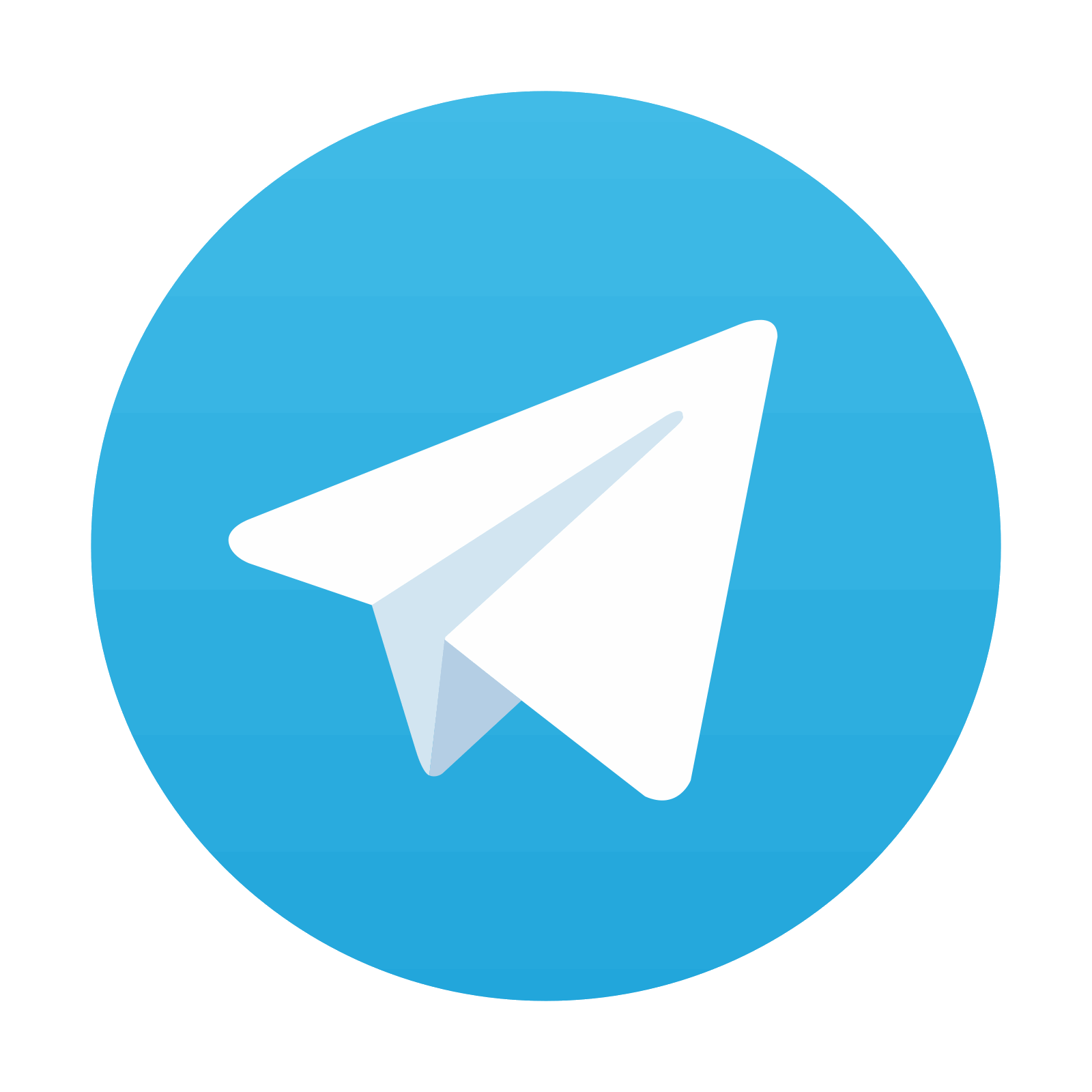
Stay updated, free articles. Join our Telegram channel
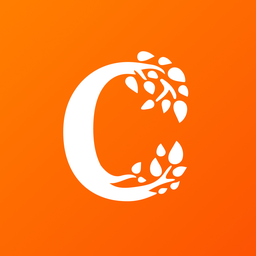
Full access? Get Clinical Tree
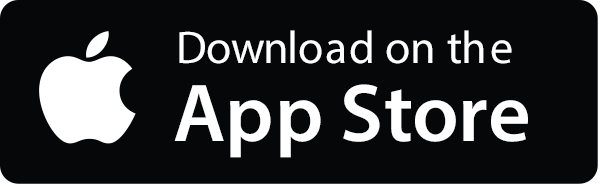
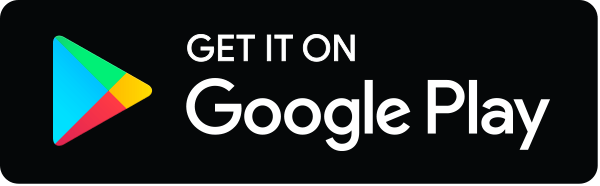