Definition
Characteristics
Clinical group(s)
Pulmonary hypertension (PH)
Mean PAP ≥25 mmHg
All
Pre-capillary PH
Mean PAP ≥25 mmHg
1. Pulmonary arterial hypertension
PWP ≤15 mmHg
3. PH due to lung diseases
CO normal or reduced
4. Chronic thromboembolic PH
5. PH with unclear and/or multifactorial mechanisms
Post-capillary PH
Mean PAP ≥25 mmHg
2. PH due to left heart disease
PCWP >15 mmHg
CO normal or reduced
Passive
TPG ≤12 mmHg
Reactive (out of proportion)
TPG >12 mmHg
The Pulmonary Vasculature
The lung has a dual blood supply from the bronchial and pulmonary circulations. While bronchial blood flow is a small part (2 %) of the left ventricular output; the pulmonary circulation carries the entire output of the right ventricle (RV) which in the absence of significant intravascular shunting equals left ventricular output and supplies the lung with the mixed venous blood draining all the tissues of the body [4]. It is the blood in the pulmonary circulation that participates in the gas exchange process.
Despite typically similar blood flow, the pulmonary and systemic circulation have major differences. The pulmonary artery and its branches have thinner walls (comprised of less smooth muscle and elastin), and greater internal diameters when compared to the systemic arteries; as well, there are no smaller denominators of pulmonary artery that correspond to the highly muscular systemic arterioles [5]. As the result of these factors the pulmonary arteries have lower resistance and are both more distensible and compressible when compared to systemic arteries (Fig. 10.1). The location of pulmonary vessels in the thorax subjects them to alveolar and intrapleural and intrathoracic pressure changes that occur with respiration [4, 5]. These characteristics combine to underscore the importance of considering transmural pressure gradient (during both spontaneous as well as mechanical ventilation) as a determinant of PVR. It is important to know during which respiratory phase pulmonary vascular pressures are measured and a standard should be followed when reporting such.
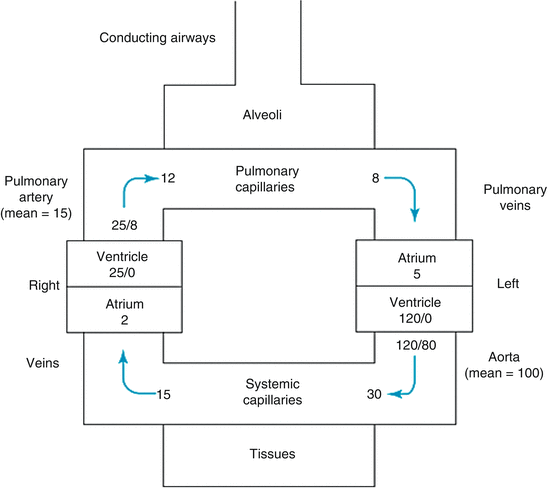
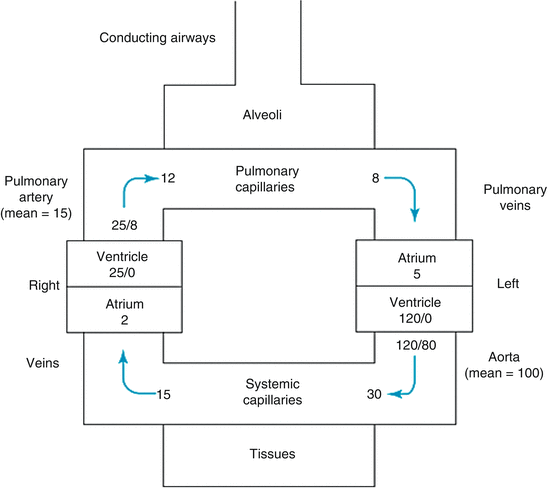
Fig. 10.1
Normal cardiac chamber pressures
Pulmonary Vascular Resistance (PVR)
PVR cannot be measured directly, but must be calculated. According to Hagen–Poiseuille’s law that assumes laminar flow, non-compressible fluid and no flow acceleration, the pressure difference across a non-distensible tube is equal to flow multiplied by resistance represented by the equation P1 − P2 = Q × R, where P1 is pressure at beginning of the tube, P2 is pressure at the end of the tube, Q is flow and R is resistance. Extrapolating this to pulmonary circulation, PVR = (mPAP − mLAP)/PBF, where PVR is pulmonary vascular resistance, mPAP is mean pulmonary artery pressure, mLAP is mean left atrial pressure and PBF is pulmonary blood flow which in the absence of significant intravascular shunting is equal to systemic cardiac output [4, 6].
As intravascular pressures, resistance, impedance, and afterload are lower in the pulmonary circulation as contrasted to the systemic arterial circulation, the left ventricle has greater metabolic demand and is thicker than the right ventricle. Under normal physiologic conditions, pulmonary arterial pressure (PAP) need not be as high as the systemic arterial pressure to overcome the effects of gravity, nor to distribute blood flow among several vascular beds. While in systemic circulation the majority of vascular resistance lies in the systemic arterioles, in the pulmonary circulation vascular resistance is evenly distributed among the pulmonary arteries, capillaries and veins. As the pulmonary circulation holds the same amount of blood with lower pressures, under normal circumstances PVR is one sixth to one ninth of the systemic vascular resistance. In addition to intrinsic vascular factors that regulate pulmonary vascular smooth muscle tone (Table 10.2), extravascular or mechanical factors (Table 10.3) are important in the regulation of PVR.
Table 10.2
Factors affecting PVR specifically pulmonary vascular smooth muscle (modified from Levitzky MG. Chapter 4. Blood flow to the lung. In: Levitzky MG. eds. Pulmonary Physiology, 8e. New York, NY: McGraw-Hill; 2013)
Increase | Decrease |
---|---|
Stimulation of sympathetic innervation (may have greater effect by decreasing large vessel distensibility) | Stimulation of parasympathetic innervation (if vascular tone is already elevated) |
Norepinephrine, epinephrine | Acetylcholine |
Alpha adrenergic agonists | B-adrenergic agonists |
PGF2A | PGE1 |
PGE2 | PGI2 |
Thromboxane | Nitric oxide |
Endothelin | Bradykinin |
Angiotensin | |
Histamine | |
Alveolar hypoxia | |
Alveolar hypercapnea | |
Low pH |
Table 10.3
Factors affecting PVR—passive or mechanical factors (modified from Levitzky MG. Chapter 4. Blood flow to the lung. In: Levitzky MG. eds. Pulmonary physiology, 8e. New York, NY: McGraw-Hill; 2013)
Factor | Effect on PVR | Mechanism |
---|---|---|
Lung volume increase above functional residual capacity (FRC) | Increases | Lengthening and compression of alveolar vessels |
Lung volume decrease above FRC | Increases | Compression of and less traction on extra-alveolar vessels |
Increased pulmonary artery pressure; increased left atrial pressure; increased pulmonary blood volume; increased cardiac output | Decreases | Recruitment and distention Long term effect of these factors could be elevation in PVR due to vascular remodeling |
Gravity; body position | Decreases in gravity-dependent regions of the lungs | Hydrostatic effects lead to recruitment and distention |
Increased (more positive) interstitial pressure | Increases | Compression of vessels |
Increased blood viscosity | Increases | Viscosity directly increases resistance |
Positive-pressure ventilation | ||
Increased alveolar pressure | Increases | Compression and de-recruitment of alveolar vessels |
Positive intrapleural pressure | Increases | Compression of extra-alveolar vessels; compression of vena cava decreases pulmonary blood flow and leads to derecruitment |
Gravitational Distribution of Pulmonary Blood Flow
Traditionally, gravity is considered to be an important factor affecting distribution of pulmonary blood flow (Fig. 10.2). In this classical model the lung is considered to have three zones: zone 1 has higher alveolar pressure than PAP and hence no blood flow occurs, in zone 3, PA and pulmonary vein pressure (PVP) are both greater than the alveolar pressure and hence blood flow occurs based on the pressure gradient between the PA and the PV, and in zone 2 PA pressure is higher than alveolar pressure and the driving force for pulmonary blood flow is the pressure difference between alveoli and pulmonary artery [7].
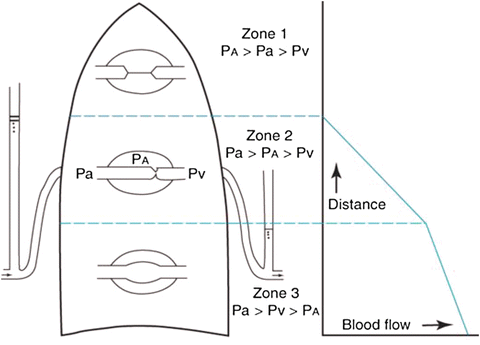
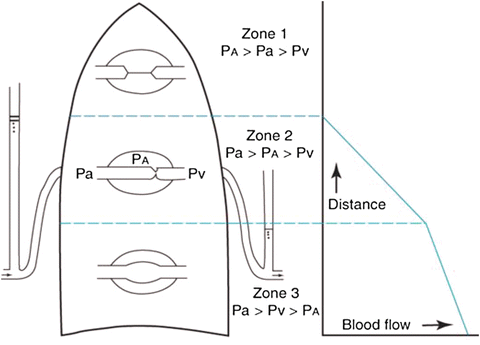
Fig. 10.2
Lung zones in gravitational distribution of pulmonary blood flow model
Although gravity has a measurable effect on pulmonary blood flow, recent studies have shown that gravity may not be the most important factor affecting distribution blood flow in lung as compared to anatomy and anatomic structure of the pulmonary arterial tree [8, 9]. While measuring PAP and PVR during catheterization this concept of zones of lungs is important to keep in mind especially if pressures are being measured in an upright patient.
Hypoxic Pulmonary Vasoconstriction
Hypoxia has important physiologic effects on pulmonary vascular tone, typically balancing less ventilated and more poorly oxygenated alveoli with reduced blood flow under normal conditions. Arteriolar vasoconstriction (Fig. 10.3) in the less oxygenated alveoli, reduces the blood flow and hence decreases contamination of the pulmonary venous blood with poorly oxygenated blood [10].
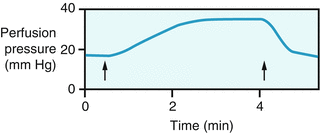
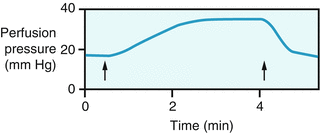
Fig. 10.3
Effect of hypoxia on vascular resistance of an isolated rat lung. The lung was perfused with blood at a constant flow. When the O2 tension of the inspired air was reduced (between the arrows), the pulmonary resistance vessels constricted, as indicated by the substantial rise in perfusion pressure
Noninvasive Hemodynamic Assessment of Pulmonary Hypertension
Clinical Examination
The noninvasive evaluation of a patient starts with clinical examination. Patients who are using pulmonary vasodilator pharmacologic therapy have a distinct facial flushing which can mask effects of systemic arterial vasoconstriction due to low systemic cardiac output (and at times can simulate distributive physiology). Use of sphygmomanometric response of systemic blood pressure to valsalva maneuver is extremely useful in estimating pulmonary capillary wedge pressure (PCWP) [11]. Inflation of brachial cuff to blood pressure 15 points higher than systemic arterial systolic blood pressure, performance of valsalva maneuver for up to 10 s and observation of phase 2 response allows for reliable and reproducible estimation of PCWP. Normally, Korotkoff sounds are heard during the initial phase of Valsalva then stop and return again at the end of Valsalva (phase 2). The persistence of Korotkoff sounds for either ≥4 beats but <10 s after the start of Valsalva, or for ≥10 s (“square root response”) predicts elevated PCWP. Jugular venous pulsations (JVP) are elevated, at times with prominent “v” waves if significant tricuspid regurgitation is present. An elevated JVP signifies an elevated RV end diastolic pressure and hence RV failure and poor prognosis. Venous hypertension can also be examined by provoking the abdominojugular reflux sign, which indicates a volume-overloaded state and limited compliance of the systemic venous system. The abdominojugular reflux sign is provoked by applying consistent pressure over the right upper abdominal quadrant, for at least 10 s. A sustained rise of >3 cm in the venous pressure for at least 15 s is a positive response [12]. It is also important to note how quickly the JVP “falls” when the abdominal pressure is removed to assess the compliance of the systemic venous system.
Clubbing may be present and is a marker of chronic hypoxemia. The RV is anteriorly positioned, directly behind the sternum and chest wall; a palpable “right ventricular” heave is variably present when it is enlarged. Auscultation may include a loud pulmonary component of the second heart sound (P2), a holosystolic murmur of tricuspid regurgitation, a diastolic murmur of pulmonary insufficiency and a third sound of RV origin. Tricuspid regurgitation in the setting of markedly elevated RV systolic pressure and an enlarged RV may produce a high-pitched murmur. Lung sounds are usually normal; “wet” crackles suggest elevated left ventricular end diastolic pressure, disease, while “dry” inspiratory crackles may point towards interstitial lung disease [13]. Hepatomegaly, elevated JVP, peripheral edema, ascites, and cool extremities are consistent with low output right heart failure, indicating more advanced disease.
Chest X-Ray
A chest X-ray cannot be used for diagnosis of PH; however, certain findings can suggest important hemodynamics even though these findings are not sensitive or specific. An increased diameter of the right descending pulmonary artery may suggest significant elevated PAP. Increased hilar thoracic index (the horizontal distance between the outer borders of the right and left pulmonary arteries divided by the maximum transverse diameter of the thoracic cage. A ratio <0.35 is considered to be normal) is also suggestive of elevated PAP [14, 15]. In a prospective study of idiopathic pulmonary arterial hypertension (IPAH), the chest radiograph demonstrated prominence of the main pulmonary artery in 90 % of patients, enlarged hilar vessels in 80 %, and decreased peripheral vessels in 50 %. All three abnormalities were seen in just over 40 % of subjects, and the presence of all three abnormalities was associated with a higher mPAP and lower cardiac index [16]. The PCWP can be estimated by observing the vascular pattern and the presence of interstitial or alveolar edema on chest radiographs. It has been suggested that PCWP greater than 13 but less than 18 mmHg indicates the presence of vascular redistribution with relative hypervascularity of the upper lung fields. When PCWP is 18–25 mmHg, interstitial pulmonary edema is seen and when greater than 25 mmHg, alveolar edema and often pleural effusion is present [17, 18]. Such changes may be absent in the presence of remodeling changes within the pulmonary vascular bed.
CT Scan
CT scan and angiography are pivotal in diagnosing pulmonary embolism and chronic thromboembolic pulmonary hypertension (CTEPH), peripheral pulmonary arterial stenosis, and mechanical or other etiologies of obstruction within the pulmonary vasculature as causes of PH (as well as for ruling out significant primary parenchymal lung disease). However, for direct hemodynamic assessment of PH, CT has a limited role. Some CT findings that can predict hemodynamics include the main pulmonary artery (MPA) caliber, segmental artery to bronchus ratio [19, 20, 22], and presence of pericardial effusion [21]. MPA caliber greater than 29 mm measured 2 cm from the pulmonary valve has 84 % sensitivity, 75 % specificity, and 97 % positive predictive value for the presence of pulmonary arterial hypertension. Also, if the MPA has a maximum transverse diameter greater than that of the proximal ascending thoracic aorta, sensitivity is 70 %, specificity 92 %, and positive predictive value 96 % for the presence of pulmonary arterial hypertension. Segmental artery to bronchus ratio greater than 1.25 times the caliber of the adjacent bronchus suggests elevation of PAP [21] and pericardial thickening or effusion is suggestive of mPAP > 35 mmHg.
MRI
The use of MRI in management of patients with PH is evolving. Phase contrast MRI calculated pulmonary vascular index, acceleration time (defined as time from onset of PA forward flow to maximum velocity), the ratio between acceleration time and RV ejection time are being evaluated to calculate PAP but results have been variable [23]. Kuehne and colleagues showed that PVR assessment using MRI-guided catheterization and MRI velocity mapping provided more reproducible results than the traditional thermodilution method. In addition, this technique seems to provide the ability to sample PVR more comprehensively (including both overall and branch-specific resistance) than can be achieved using Doppler guidewires [24].
Electrocardiogram
Electrocardiogram (ECG) lacks sensitivity and specificity in diagnosing PH. In patients with PH, sinus tachycardia may be the only abnormality present (EKG could be completely normal). RV strain, right ventricular hypertrophy (RVH), incomplete right bundle branch block and increased P wave amplitude are common findings [25]. On occasion, in patients in whom PAH attenuating treatment has been very effective, dramatic ECG changes from a pattern corresponding with RVH to a (near)-normal pattern have been reported [26]. P wave amplitude has been shown to predict prognosis in PAH [27].
Echocardiogram
The transthoracic echocardiogram (TTE) remains a core element of RV assessment with applications in the initial and longitudinal diagnosis and screening of pulmonary hypertension, discrimination between contributors to etiology, as well as in assessment of RV and valvular function. The European Society of Cardiology guidelines for the diagnosis and treatment of PH suggest the following: (1) PH is unlikely for tricuspid regurgitation velocity (TRV) ≤2.8 m/s, systolic PAP (sPAP) ≤ 36 mmHg (assuming RAP of 5 mmHg), and no additional echocardiographic signs of PH; (2) PH possible for TRV ≤ 2.8 m/s and sPAP ≤ 36 mmHg, but the presence of additional echocardiographic signs of PH or TRV of 2.9–3.4 m/s and SPAP of 37–50 mmHg with or without additional signs of PH; and (3) PH likely for TRV > 3.4 m/s and sPAP > 50 mmHg with or without additional signs of PH [3]. Combined with well-validated hemodynamic calculations, focused TTE can provide very close approximations of the pulmonary vascular and RV hemodynamics.
Systolic Pulmonary Artery Pressure (sPAP)
TTE-based estimation of sPAP most frequently relies upon determination of Doppler-based peak TVR and use of the modified Bernoulli equation (∆pressure = 4 × velocity [2]) to approximate differences between RV and right atrial (RA) pressure. Further addition of TTE-guided estimation of RA pressure (RAP) allows for calculation of RV systolic pressure (which in the absence of obstruction to RV outflow represents sPAP). As central venous pressure (CVP) and RAP are essentially equivalent in the absence of anatomic obstruction, the latter is commonly evaluated by measuring the size, flow patterns and the respiratory variation of the inferior vena cava (IVC). Generally, IVC diameter <2.1 cm and collapse greater than 50 % on inspiration would correspond to a normal RAP of 0–5 mmHg. The presence of either greater diameter or lesser collapse (but not both) correspond to RAP of 5–10 mmHg, and a diameter greater than 2.1 cm with less than 50 % collapse corresponds to a high RAP of 10–20 mmHg [28, 114]. These criteria are relatively poor indicators of RAP, however, and this should be acknowledged when estimating PAP [29]. Patients who cannot comply with taking a deep inspiration to demonstrate collapsibility of the IVC can be asked to perform a “sniff” maneuver, which causes a decrease in intrathoracic pressure.
Although widely accepted as a screening test, the precision of this echocardiographic estimate of PAP is modest. In studies that have compared echocardiographically estimated values and values measured by right heart catheterization (RHC), the mean difference ranged from 3 to 40 mmHg, and sPAP was underestimated with the echocardiographic method by >20 mmHg in 31 % of patients studied [30]. To minimize error in acquisition of the TR envelope by Doppler, it is recommended that TRV be measured in multiple views and the maximal velocity jet found should be used for the calculation. Inadequate regurgitant signals can be enhanced with the use of contrast. It should always be remembered that sPAP evaluation with Doppler methods, however, cannot definitively diagnose PH and has limited role in the decision to treat patients or to monitor therapy efficacy.
Mean Pulmonary Artery Pressure (mPAP)
The simplest method of calculating the mPAP may be by using sPAP as follows:
As the only variable in the above equation is sPAP, this calculation carries the same pitfalls as measurement of sPAP mentioned above.
Doppler-based measure of diastolic pulmonary valve regurgitation may be difficult to obtain, and therefore may be absent on particular studies. When present and complete, use of the peak pulmonary valve regurgitation velocity (typically early in diastole) in the modified Bernoulli equation allows estimation of peak pressure difference between RV and PA in early diastole. The peak pulmonic regurgitation velocity represents the diastolic pressure gradient between the pulmonary artery and the RV. Adding the RAP to such calculation often provides acceptable estimation of mPAP (mPAP = 4 × (peak pulmonic valve regurgitation jet velocity)2 + RAP) [32].
RV–RA Mean Systolic Gradient
When TTE-based estimation of RAP is added to TR Doppler-velocity based approximation of peak systolic RA–RV systolic pressure difference, a value is obtained that has excellent correlation with mPAP measured during an invasive RHC [33]. Critical to use of this methodology is capture of complete and accurate tricuspid regurgitation Doppler envelope.
Diastolic Pulmonary Artery Pressure (dPAP)
Application of the modified Bernoulli equation to the end-diastolic pulmonary regurgitation velocity allows estimation of the end-diastolic gradient between the RV and PA, which, when added to the TTE-based estimation of RA pressure, estimates diastolic PA pressure, (dPAP), with a high correlation with invasive dPAP measurements [34]. Similar estimation can be obtained by Doppler-based assessment of the earliest systolic gradient at the time of pulmonary valve opening (closely approximating end-diastolic gradient between RV and PA) and adding TTE-based estimation of RA pressure, yielding estimate of dPAP [35, 36].
Pulmonary Vascular Resistance
Accurate assessment of PVR provides an understanding of the mechanism of elevated PAP and aids in selecting therapy for PH. As a result of its clinical importance, numerous TTE-based estimations of PVR have been developed. Noninvasively, one can relate trans-pulmonary gradient (TPG) to pulmonary blood flow by determining the ratio between the tricuspid regurgitation velocity and the velocity time integral of the pulmonary flow at the RV outflow tract. This equation simplifies to:
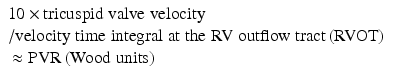
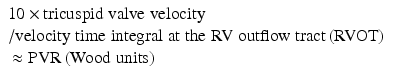
Individuals with ratios <0.2 have been demonstrated to likely have low PVR values (<2 WU), with 70 % sensitivity and 90 % specificity, even in the presence of increased Doppler sPAP [37].
Alternatively, relating sPAP by the heart rate-corrected velocity time integral (VTI) at the RVOT, namely sPAP/(HR × VTIRVOT), also estimates PVR but takes into account RAP and heart rate (cutoff value of 0.076 with >80 % sensitivity and specificity in a population with PVR > 15 Wood units) [38, 39].
Additional analysis of the pre-ejection systolic time (the period of the cardiac cycle between onset of tricuspid regurgitation and beginning of elevation of PAP) related to systolic acceleration time (period of time between the beginning of ejection and peak flow velocity), normalized for total systolic (ejection and non-ejection) time linearly correlates with invasively measured PVR between 0 and 9 Wood units [40].
An additional technique to estimate PVR is based on the fact that mid systolic notching of the RVOT envelope relates to pathologic wave reflection in the setting of elevated pulmonary artery impedance and PVR of at least 3 Woods units [41]. The ratio of sPAP Doppler to RVOT tract VTI with or without a constant value of 3 designates presence of RVOT VTI midsystolic notching. {PASP/RVOTVTI + 3 if midsystolic notching of RVOT envelope is present} provides superior agreement with catheterization estimates of PVR across a wide range of values.
Morphology of the Doppler Velocity Curves
The Doppler pulmonary flow velocity curve has a dome-like appearance in subjects with normal pulmonary pressures; this is transformed to a somewhat “triangular” shape in patients with PH. Acceleration time (AT) is decreased in the presence of elevated sPAP and mPAP, with peak velocity appearing earlier in systole. An AT <93 ms correlates well with invasive PAP elevation [42, 43]. At times of concomitant abnormality of PA compliance, capacitance or PVR, a second slower rise in velocity during mid-systolic flow deceleration can be observed, resulting in so-called “midsystolic notching” [44].
The combination of assessment of presence or absence of mid systolic notching of the RVOT envelope with estimate of Doppler acceleration time is one of the most utilized echocardiographic techniques to determine if PVR is elevated.
Estimates of RV Function
In response to pressure overload, the RF typically dilates and develops concentric hypertrophy. The TTE-based apical “4-chamber” view allows an expansive view of the heart. Transition of the RV from a triangular to a more globular or rounded shape can be demonstrated. Similarly as the RV expands in size, it may replace the LV in forming part or all of the cardiac apex. While quantification of the size of the RV remains a limitation of echocardiography, the ratio between the diastolic inflow diameters and the areas of the RV and the LV (0.6–1 mild, >1 severe) appear to correlate well with the degree of RV dilatation [45].
More complex methodology requires tracing of RV endocardium during systole and diastole to calculate the fractional are change (FAC) (RVFAC = (end-diastolic area – end-systolic area)/end-diastolic area × 100, normal being ≥35 %) as a correlate of RV systolic function [28, 46].
One of the most utilized indices of RV contractile function is perhaps one of the simplest to obtain. The longitudinal tricuspid annulus plane systolic excursion or TAPSE is calculated via M-mode echocardiography (though two-dimension estimates are often performed) of the right ventricular annulus. Normal values are >2.0 cm and are reduced in case of RV dysfunction [47]. Important limitations to this method include its dependency on proper image acquisition angle and RV load dependency, its focus on a small part of the RV myocardium, independent changes that occur after surgical or other penetration of the pericardium, and the fact that the tricuspid annulus motion may be very well affected by the overall heart motion [48]. TAPSE values of less than 1.8 cm correlate with worse 1 and 2 year prognosis in patients with groups 1 and 2 PH.
While the myocardial performance Tei index (sum of isovolumetric contraction time and isovolumetric relaxation time indexed to the total LV ejection time [(IVCT + IVRT)/(LVET)]) has been correlated with invasive hemodynamics and clinical outcomes, its use in care practice has been limited [49]. Additional complex hemodynamic assessments from TTE include tissue Doppler and strain assessment. Current American Society of Echocardiography guidelines regarding right-heart assessment recommend that basal RV free wall S′ < 10 cm/s should be considered a marker of RV dysfunction [28]. Mitral annular velocity should be checked to consider the presence of elevated left-sided filling pressures. Strain imaging can suggest RV dysfunction in the presence of decreased RV longitudinal strain and impairment of left ventricular segmental longitudinal and circumferential strain (when greater for the interventricular septum than for the LV free wall) [50].
The “reverse Bernheim effect” suggests RV and LV interaction such that poor RV function in the face of marked afterload can worsen LV filling and output [51]. The ratio of the long axis to short axis diameters of the left ventricle, both in diastole and in systole, otherwise known as the “eccentricity index” assesses LV compression, differentiates between volume and pressure overload, and is assessed on the two-dimensional short-axis view of the LV [52]. In addition, RV dilatation also causes abnormal left ventricular filling which may be assessed by evaluating the Doppler appearance of the mitral inflow. Abnormal relaxation pattern is often a surrogate to LV cavity compression in patients with more severe forms of PH.
The presence of pericardial effusion may be due in part to poor pericardial drainage in the setting of RA hypertension and lower cardiac output. While presence of effusion is a poor prognostic finding, tamponade is not a typically encountered clinical sequelae due to the high pressure in the RA and RV [53].
Right to left intravascular shunting (and resultant hypoxemia unresponsive to oxygen administration) through a patent foramen ovale can be demonstrated with agitated saline or echocardiographic contrast injection, and may be aggravated in the setting of elevation of RA pressure either at rest or with exercise,.
Right Heart Catheterization
Invasive hemodynamic assessment of PH is a dynamic process that often requires continuous recordings of multiple variables and thus, the need for RHC. For the assessment of PH, RHC typically includes vasoreactivity testing and may include exercise and fluid challenge as well as angiography. RHC remains essential for accurate diagnosis of most forms of PH including PAH. Standard of care practice guidelines strongly recommend that patients suspected of having PH after noninvasive evaluation undergo RHC prior to initiation of therapy.
Indications
In the evaluation of patients with PH, RHC is usually indicated for the following [2, 3]
(a)
In all patients with PAH to confirm the diagnosis, to evaluate severity and to assist in selection of PAH specific therapy.
(b)
For confirmation of efficacy of PAH-specific therapy.
(c)
For confirmation of hemodynamic effects of suspected clinical deterioration and as baseline for the evaluation of the effect of treatment escalation and/or combination therapy.
RHC may have limited benefit when an alternative diagnosis is obvious on non-invasive testing. Similarly, RHC may have specific but limited use when elevated PAP pressures on non-invasive testing can be explained by left heart disease, diastolic dysfunction, or chronic lung disease.
Table 10.4 lists the essential components of invasive hemodynamic assessment of PH. The addition of exercise testing, vasoreactivity testing or fluid challenge, and angiography to RHC is discussed in appropriate sections below.
Oxygen saturations (SVC, IVC, RA, RV, PA’s, SA) |
Right atrial pressure |
Right ventricular pressure |
Pulmonary artery pressure, systolic, diastolic, mean |
Pulmonary arterial wedge pressure, left atrial pressure, or left ventricular end-diastolic pressure |
Cardiac output/index |
Pulmonary vascular resistance |
Systemic blood pressure |
Heart rate |
Response to acute vasodilator |
Indications for Coronary Angiography with Right Heart Catheterization for Evaluation of Pulmonary Hypertension
It is not infrequent that assessment of coronary anatomy may carry import implications when performed as part of RHC to evaluate PH. The reasons for such include, but are not limited to, evaluation of the coronary anatomy as part of surgical planning, presence of coronary-fistula to the right-sided chambers as a possible source of left to right shunt, presence of atherosclerotic coronary artery disease as a possible reason for impaired LV function or elevated filling pressure, and evaluation of the anatomic relations between the coronary arteries and the pulmonary arteries for specific procedures (e.g., percutaneous prosthetic pulmonary valve implantation).
Safety
RHC, although technically demanding, is a safe procedure when performed by experienced operators [54]. Hoeper and colleagues reported on 5,727 RHC procedures retrospectively and 1,491 prospectively, for a total of 7,218 procedures. The overall number of serious adverse events was 76 (1.1 %, 95 % confidence interval 0.8–1.3 %). The most frequent complications were related to venous access (e.g., hematoma, pneumothorax), followed by arrhythmias and hypotensive episodes related to vagal reactions or pulmonary vasoreactivity testing. The vast majority of these complications were mild to moderate in intensity and resolved either spontaneously or after appropriate intervention. Four fatal events were recorded in association with any of the catheter procedures, resulting in an overall procedure-related mortality of 0.055 % (95 % confidence interval 0.01–0.099 %). Of the four fatal complications only two were related to the procedure itself (PA rupture and both electromechanical dissociation and intrapulmonary hemorrhage) [55]. Adequate patient preparation such as management of anticoagulation issues can help in reducing vascular access complications. It should be remembered that RHC as an elective, planned procedure is a very safe procedure and is very different from placement of a pulmonary artery catheter (PAC) in patients admitted to the intensive care unit (ICU) where the use of PAC has, in some studies, been associated with increased mortality. Also while a PAC placed in an ICU patient may stay in situ for several days, thus increasing vascular, infectious, and thromboembolic complications, diagnostic RHC is typically a short outpatient procedure lasting under an hour.
Patient Preparation
Policy regarding fasting status of patients, use of peripheral IV, and withholding of particular medications (such as hypoglycemics, vasodilators, diuretics, or anticoagulants) should be dependent upon individual laboratory preference, health status of the patient, and whether sedative use is planned. A clear plan for such though, should be constructed and documented. For example, the calculation of risk-benefit ratio of sustaining therapeutic INR up until catheterization for an individual patient in whom anticoagulation has been prescribed for mechanical mitral valve and atrial fibrillation may well be different than that of another patient in whom warfarin is prescribed for primary prevention of stroke with atrial fibrillation. Clear description of such logic should be available to personnel within the team assisting in catheterization (Table 10.5).
Table 10.5
Anticoagulation management in case decision is made to withhold anticoagulation (modified from Massicotte A. A practice tool for the new oral anticoagulants. Can Pharm J (Ott). Jan 2014) [56]
Drug and creatinine clearance | Recommendation in preprocedure period | Recommendation in postprocedure period | Bridging with heparin needed |
---|---|---|---|
Warfarin | Stop 48 h prior until INR <1.7 (varies per institutional policy). | Resume therapy when hemostasis is adequate and clinical condition allows | Needed if moderate to high risk of thromboembolism |
Dabigatran ≥80 ml/min | Stop 24 h before surgery. | Resume therapy when hemostasis is adequate and clinical condition allows | Needed only if patient cannot take oral medications and there is moderate to high risk of thromboembolism |
Dabigatran 50 to <80 ml/min | Stop 1–2 days before surgery. | Resume therapy when hemostasis is adequate and clinical condition allows | Needed only if patient cannot take oral medications and there is moderate to high risk of thromboembolism |
Dabigatran <30 ml/min | Stop at least 5 days before surgery. | Use alternative anticoagulation agent | NA |
Rivaroxaban ≥30 ml/min | Stop at least 24 h before surgery. | Resume therapy when hemostasis is adequate and clinical condition allows | Needed only if patient cannot take oral medications and there is moderate to high risk of thromboembolism |
Rivaroxaban <30 ml/min | Last dose on day −3. | Use alternative anticoagulation agent | NA |
Apixaban >50 ml/min | Stop at least 24 h before surgery. | Resume therapy when hemostasis is adequate and clinical condition allows | Needed only if patient cannot take oral medications and there is moderate to high risk of thromboembolism |
Sedation
While concern has been raised regarding safety and influence of sedatives used during catheterization on medical status and physiologic indices including loading conditions and inotropy, this is probably of minor concern unless liver and kidney functions are significantly impaired [57–59]. Nonetheless, it is becoming more common practice to avoid standard use of systemic sedation prior to or during RHC.
Right Heart Catheterization in a Mechanically Ventilated Patient
Complex influences by mechanical ventilation must be considered when assessing measured hemodynamics. Positive pressure ventilation, particularly in the setting of PEEP, variably increases RV afterload with resultant decline in RV contractility, and can contribute to elevated measure of PCWP particularly during inspiration [60, 61]. Additional influences may include baseline vascular and myocardial loading conditions and compliance of the chest wall and lungs. General practice is to discount meaningful influence of PEEP <10 cm H2O on measured PCWP, with correction of PCWP when PEEP ≥10 cm H2O by 2–3 cm for every 5 cm H2O increment in PEEP [62, 63]. Another cause of falsely elevated PCWP in a mechanically ventilated patient is the monitoring catheter being located outside zone 3 (during positive pressure ventilation less of the lung is zone 3 due to elevated alveolar pressures). Care should be taken to demonstrate catheter tip placement within zone 3, gravitationally below the level of the left atrium [64].
Hemodynamic pressure measurements should reflect the time of most neutral intrathoracic pressure (in spontaneously respiring patients this occurs at end expiration, with converse in patients utilizing positive pressure ventilation), rather than sole recording of mean pressure. Esophageal manometry is rarely utilized to estimate the effects of high swings of intrathoracic pressure seen with labored breathing. When possible, the patient should be instructed to expire slowly to allow pressure recording that may assist in accuracy of hemodynamic measure [72].
Percutaneous Access Sites
Commonly used access sites include internal jugular (IJ) vein, femoral vein, brachial vein, and subclavian vein. Knowledge of prior procedures, venous and arterial obstructions, compressions, complex anatomical course and prior procedural complications is necessary to choose access site in an individual patient. The IJ vein is usually the preferred site because of its ease of access, lower complication rate and because it allows patients to be discharged early after procedure [54, 55, 65]. Proximal arm veins provide ready access to RHC, but catheter manipulation may be difficult and catheter size choice is limited. Some operators may prefer femoral vein if concomitant left heart catheterization is planned via femoral artery. Ultrasound guidance should be considered for both IJ and femoral access sites. Patients with kidney disease, congenital heart disease, pacemakers, or prior indwelling venous access for other reasons (e.g., TPN) are more likely to have had multiple prior procedures and consequent vascular injury or thrombosis. Imaging of the vascular bed may be established by various modalities (ultrasound, computed tomography and magnetic resonance angiography) and should be considered by the operator when deemed appropriate. The use of a micropuncture kit with a 21-gauge needle and introducer can minimize potential trauma from inadvertent puncture of the carotid artery. Table 10.6 lists the advantages and disadvantages of different catheterization access sites.
Table 10.6
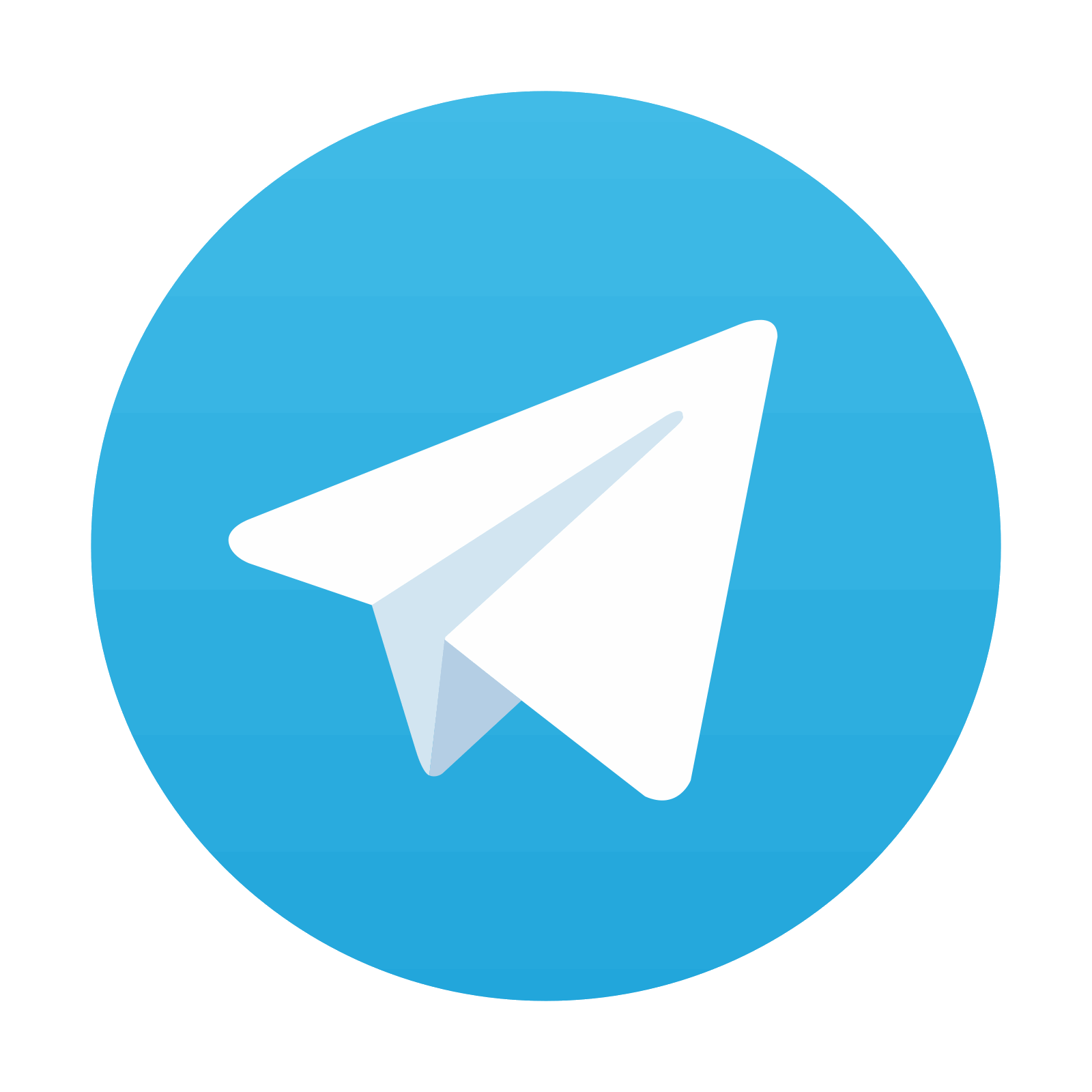
Access sites for right heart catheterization
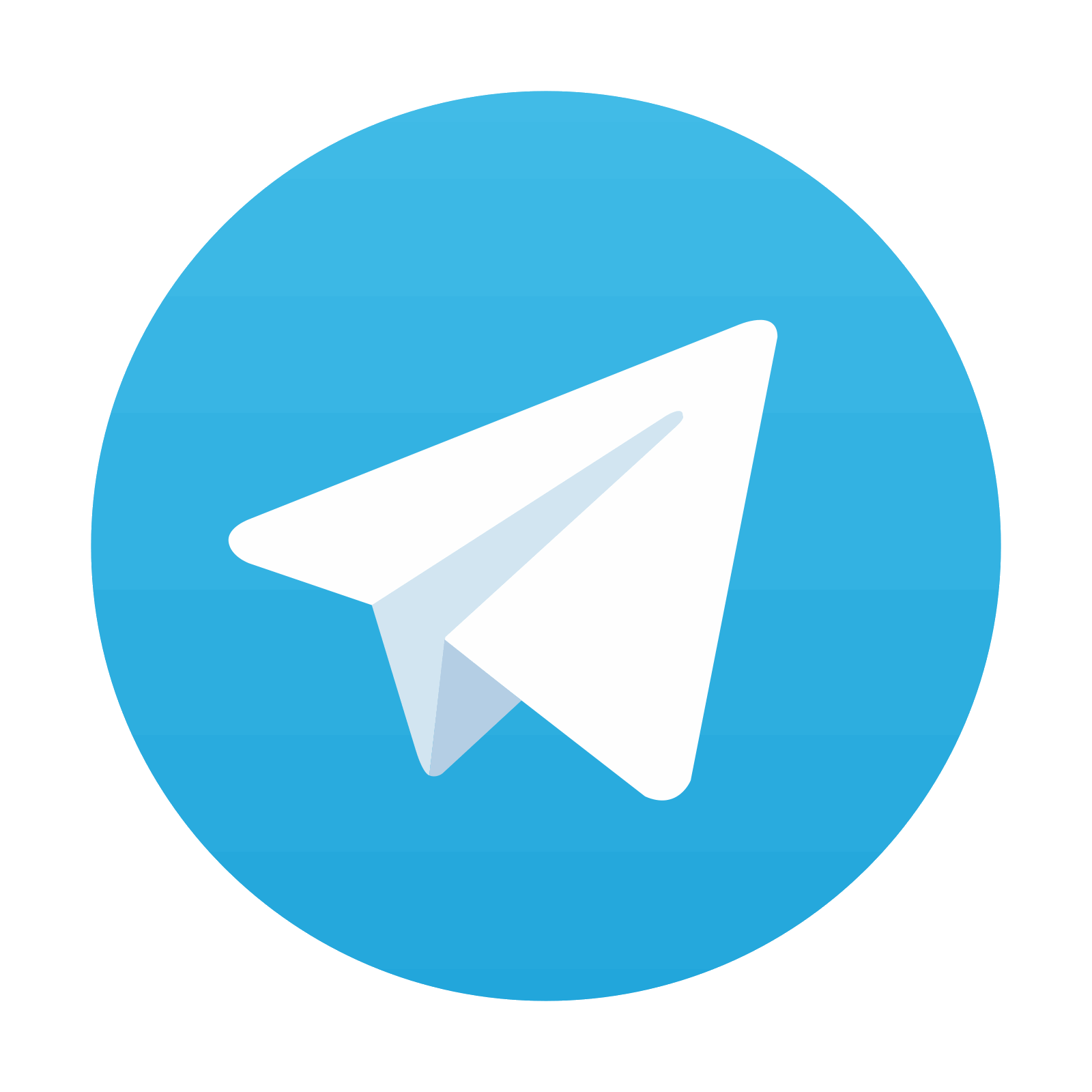
Stay updated, free articles. Join our Telegram channel
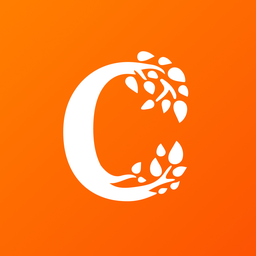
Full access? Get Clinical Tree
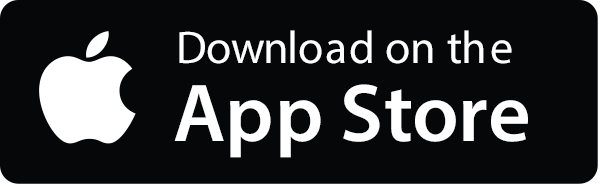
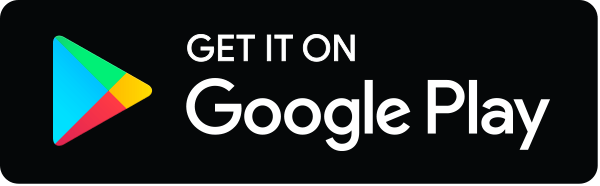
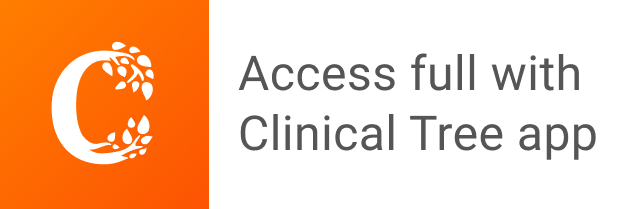