Understanding the features of the pulmonary circulation is critically important in the management of patients with congenitally malformed hearts. The past 10 years have seen remarkable advances in our understanding of its development, the genetics, pathobiology, and treatment of pulmonary vascular disease, and the physiology of this circulation. Primary intracardiac repair in infancy has become the norm, but the selection of patients suitable for intracardiac repair can still pose a problem, particularly in developing countries when many patients present late. Assessment has been facilitated by improvements in tissue Doppler imaging, computerised tomography, and magnetic resonance imaging. The patients who, in the absence of surgical correction, develop the Eisenmenger syndrome and become severely symptomatic can be helped by treatment with new medicines used to treat idiopathic pulmonary hypertension. Advances in our understanding of the pathogenesis of pulmonary vascular disease now influence our approach to the management of many patients with congenitally malformed hearts and pulmonary hypertension. In this chapter, we will focus on the normal and abnormal development of the pulmonary circulation, neonatal circulation, the hypoperfused lung, and advances made in the pathobiology and treatment of pulmonary vascular disease.
NORMAL PULMONARY CIRCULATION
Normal Anatomy of the Pulmonary Circulation
The functional unit of the lung is the acinus. An acinus is all the lung tissue supplied by a terminal bronchiolus. In the mature lung, it includes up to eight generations of respiratory bronchioluses, alveolar ducts, and the alveoluses beyond ( Fig. 7-1 ). It is the respiratory unit of the lung. At the end of any airway, three to five acinuses are grouped to form a lobule. Each small airway is accompanied by a branch of the pulmonary artery until the arteries finally enter the capillary network of the alveolar walls. The acinuses and lobules, with their accompanying arteries, are the last part of the lung to appear before birth. It is these structures that are remodelled in the normal lung with growth, and which change most readily in disease. The bronchopulmonary segment is the basic topographical unit of the lung. It is a wedge of lung tissue supplied by a principal branch of a lobar bronchus and its accompanying pulmonary artery. The pulmonary veins and lymphatics lie in the intersegmental plane, receiving tributaries from adjacent segments.

The bronchopulmonary segment is the surgical unit of lung tissue, since a segment can be resected along its intersegmental boundaries. Familiarity with normal bronchopulmonary segmental anatomy also helps the cardiologist because, when examining pulmonary angiograms, he or she must ensure that all the bronchopulmonary segments are normally connected to the intrapericardial pulmonary arteries. Segments that are not perfused by a normal pulmonary arterial supply must be identified, and a search made for their source of systemic arterial supply. On angiography, large collateral systemic arteries arising from the aorta, often called major aortopulmonary collateral arteries, are distinguished from large bronchial arteries by their origin, distribution, and proximal connections with the hilar, lobar, or segmental arteries. The final destination of bronchial arteries is not seen angiographically, since the majority of them connect with the intra-acinar pulmonary arteries.
Bronchial Circulation
In children with congenitally malformed hearts, the bronchial circulation may provide an alternative source of arterial supply to the pulmonary capillaries when there is little or no flow of blood down the lobar or segmental arteries. In the presence of obstructed or absent pulmonary veins, the bronchial veins drain oxygenated blood from the lung into the systemic veins.
The main bronchial arterial supply usually arises from the aorta directly, or from an intermediary intercosto-bronchial artery. The number of vessels arising from the aorta to supply each lung varies in the normal circulation. The most common anatomical patterns seen in one series included two posterior bronchial arteries supplying each lung in about one-third, two arteries supplying the left and one the right lung in one-fifth, with this relationship mirror-imaged in a further sixth, and a single trunk supplying each bronchus in a further tenth. 1 The successive branches of each bronchial artery are described as superior, middle, and inferior, this reference made to the relative levels of origin from the aorta, and not the ultimate level of distribution within the lung.
Additional accessory bronchial arteries may arise from the subclavian, brachiocephalic, or internal thoracic arteries. When the bronchial arterial circulation is expanded to provide an alternative source of blood supply to the lung, these and other vessels enlarge, distributing blood through the bronchial arteries via the pulmonary ligaments and retroperitoneal tissues. Blood is also distributed from the pericardiophrenic, oesophageal, and other mediastinal vessels, and occasionally from a coronary artery. Within the lung, each lobar and segmental bronchus is accompanied by two large bronchial arteries, which frequently anastomose around the bronchus.
Microscopically, the bronchial arteries are readily distinguishable from the pulmonary arteries by their position in the lung and by their mural structure. They accompany the bronchuses to supply the adventitial and mucosal layers, along with the walls of large pulmonary arteries. They lie next to the bronchial veins and the nerves that supply the large airways. As in other systemic arteries, the medial wall is thick and muscular, the internal elastic lamina is well defined, and the external elastic lamina is interrupted or absent. The structure of bronchial arteries frequently changes with age in the normal lung. Longitudinal muscle bundles develop, which may even occlude the lumen. The mechanism for this change is not understood.
There are two types of bronchial veins: the deep and the superficial, or pleurohilar, veins. Both types communicate freely with the pulmonary veins. The deep veins originate in the walls of the terminal bronchioluses, and eventually drain into the left atrium directly or via the pulmonary veins. The pleurohilar veins from the right lung drain into the azygos vein, while those from the left lung drain into the hemiazygos, superior intercostal, or brachiocephalic veins. Microscopically, a bronchial vein has a thinner wall than a pulmonary vein for a given diameter. Unlike pulmonary veins, bronchial veins contain delicate bifoliate valves, which are more commonly seen in the immature than in the mature lung.
Microscopic Assessment of the Pulmonary Arteries and Veins
In the normal child, the lung is remodelled with growth. In the abnormal lung, therefore, each structural feature must be compared with that of age-matched controls in tissues prepared and analysed in the same manner. To make such a comparison, the intrapulmonary arteries must be identified. They cannot be identified according to their size, because this changes in the normal lung with age, and may also change in disease. In a postmortem specimen, the elastic and large muscular arteries are identified by counting the number of generations that separate them from the segmental hilum, counting the segmental artery as the first generation. Their mural structure is determined by their position in the lung. Within the acinus, arteries continue to branch with the airways. On microscopic examination of both biopsy and postmortem tissue, the arteries are identified according to the type of airway they accompany, that is, terminal or respiratory bronchioluses, alveolar ducts, or ones that lie within the alveolar walls (see Fig. 7-1 ). Describing any part of the pathway in terms of arterioles is best avoided, because this term is open to different interpretations based on size, structure, and function, or a combination of attributes. In a large and unselected population of arteries, the mean percentage medial thickness can be calculated from measurements of medial thickness and external diameter. Other assessments include the mean ratio of arterial lumen to wall, the area of muscle present, and an index of medial muscular tissue present in the lung. Population counts reveal the relationship between mural structure, size, and the position in the arterial pathway. Arterial muscularity is usually assessed by determination of percentage medial thickness, and by finding the extent to which muscle has differentiated along the arterial pathway as judged both by the size and the position of the artery in the lung field. The number of arteries per unit area, or volume, of lung can be determined and the ratio of alveoluses to arteries calculated to overcome any difference in the degree of inflation in different lungs. In postmortem specimens, the effect of hypoperfusion on lung growth can be studied by determining the number and size of alveoluses. The veins are studied in a comparable manner.
Anastomoses Between Pulmonary and Bronchial Circulations
In the normal adult, most of the proximal connections between the pulmonary and bronchial arteries accompanying the same airway occur at the level of non-cartilaginous bronchioluses. More peripherally, the small bronchial arteries become indistinguishable as they anastomose with small pulmonary arteries around the necks of respiratory and terminal bronchioluses. In addition, branches of the bronchial arteries that accompany large and small airways anastomose with adjacent thin-walled pulmonary arteries in the surrounding lung tissue. These connections are called bronchopulmonary arteries. They are relatively common in the normal fetal and neonatal lung. Subepithelial bronchial arteries also anastomose with small precapillary pulmonary arteries.
The frequency with which the bronchial and pulmonary arterial circulations anastomose explains why accidental surgical interruption of a pulmonary artery, or ligation of a collateral artery providing the only source of blood supply to a region of lung, does not usually lead to infarction of the lung. By contrast, experimental stripping of the bronchial arteries at the hilum leads to necrosis of the bronchial wall near the hilum. In the venous circulation, there are many connections between bronchial and pulmonary veins. These enlarge in the presence of pulmonary venous hypertension. Blood may then flow from the pulmonary veins indirectly to the right atrium. Arterio-venous connections are not found in the normal lung.
Pulmonary Lymphatics
The superficial lymphatic network lies in the pleura, and the deep network lies in the interstitium. The two networks anastomose around the hilum, and drain to the hilar and tracheobronchial lymph nodes. Lymphatics are present in the pleura, in connective tissue septums, around the pulmonary arteries and veins, and in the bronchial wall, including the mucosa. Microscopically, small lymphatics consist of endothelial cells lying on a basement membrane, while in the large channels the walls are strengthened by the addition of collagen and elastic fibres, and an occasional muscle cell. The lymphatics contain delicate bicuspid valves. When pulmonary venous return is obstructed, either at the mitral valve or within the pulmonary veins themselves, the lymphatics become dilated. In the presence of long-standing obstruction, the walls of the lymphatics may be thickened by an increase in the amount of smooth muscle.
Biopsy of the Lung
A biopsy should be taken from the inflated lung. It is fixed in inflation to ensure distention of the airways, and hence their identification. The biopsy will be representative of the entire vasculature in a young child, providing that the flow of blood is evenly distributed. The lingula is best avoided, since even in the normal lung the arteries of this region tend to be more thick walled. In adults with rheumatic mitral stenosis, the lower lobe vessels are usually more severely affected than the rest of the vasculature. In any biopsy, the mural structure of the pulmonary arteries varies, as is to be expected, since the pulmonary arterial tree is a branching system. All arteries at a certain point along the pathway, in both normal and abnormal lungs, are structurally similar to, and different from, arteries proximal and distant to them.
Morphologic Assessment of Right Ventricular Hypertrophy
In the normal heart, the septum is considered as part of the left ventricle. Hypertrophy of the right ventricle, therefore, is indicated by a decrease in the ratio of the weight of the left ventricle and the septum assessed relative to the weight of the right ventricle. During fetal life, the right ventricle forms a greater proportion of the total ventricular weight than it does in the child or adult. At sea level, the ratio of between 2.3 to 1 and 3.3 to 1, as expected in the adult, is normally achieved when the infant reaches 4 months of age. 2
DEVELOPMENT OF THE HUMAN PULMONARY VASCULATURE
Structural Development
Morphological study of the development of the pulmonary vasculature in the human describes the creative process, and provides clues about regulatory mechanisms which can be explored in other species and in experimental models. Abnormal development is informative in that it can be viewed as a vicarious experiment.
The heart tube is formed by the end of the third week of gestation, and about 5 days later the lung bud develops at the caudal end of the laryngotracheal groove. The lung bud expands laterally and divides into the two primordial lung sacs, each of which then divides first into lobes, and then into bronchopulmonary segments. In the normal lung, recent work shows that the pre-acinar arteries and post-acinar veins form by vasculogenesis from the splanchnopleural mesoderm of the lung bud. 3,4 The pre-acinar airways and post-acinar veins form in a centrifugal manner from hilum to periphery, but the vessels do not. 3,4 They form by continuous coalescence of endothelial tubes alongside the newly formed airways, which appear to provide a template for pre-acinar arterial development ( Fig. 7-2 ). The continuous addition of endothelial tubes gradually lengthens the pulmonary arteries and veins. The heart is connected to the two lung buds from a very early stage of embryonic life. By at least 34 days of gestation, serial reconstructions demonstrate physical continuity between the aortic sac, the pulmonary arteries, the peribronchial capillary plexus, the pulmonary veins, and the atrial component of the heart. Thus, blood can circulate through the lung earlier than had been supposed, exposing the developing structures to circulating factors generated at distant sites, including angioblasts and other cells which could become incorporated into the vessel wall. The extent to which angiogenesis contributes to formation of vessels during this pseudoglandular phase is uncertain, but it may be responsible for the supernumerary vessels. Angiogenesis predominates later, and is thought to be responsible for formation of intra-acinar arteries, which starts in the canalicular phase with further development of airways and alveoluses, and continues after birth. All the pre-acinar arteries and airways have formed by the 16th week of gestation. Between a third to a half of the adult complement of alveoluses and intra-acinar arteries form before birth, with new structures then forming most rapidly within the first 6 months of life. After 3 years of age, development mainly consists of an increase in size of existing structures.

The pulmonary arterial smooth muscle cells are derived from three sites in a temporally distinct sequence 3 ( Fig. 7-3 ). The first cells to surround the endothelial tubes migrate from the bronchial smooth muscle around the neck of each terminal bud. These cells become surrounded by the second population, which differentiates from mesenchymal cells. The third source is from endothelial cells in the most peripheral arteries, which transdifferentiate into smooth muscle cells later in gestation, from 98 to 140 days. Venous smooth muscle does not derive a contribution from bronchial smooth muscle. 4 Irrespective of their origin, all smooth muscle cells follow the same sequence of expression of smooth muscle specific cytoskeletal proteins. Maturation takes place from the subendothelium to the adventitial layers, and from hilum to periphery. In the pulmonary veins, the cytoskeletal proteins appear in the same sequence as in the pulmonary arteries, but do not express caldesmon, which might help explain why pulmonary veins are so responsive to contractile agonists.

The lymphatics originate at the hilum, and are first seen at about 56 days of gestation. 3 By the end of the pseudoglandular period, lymphatic channels have subdivided the lung periphery, positioned in the prospective connective tissue septums. The bronchial arteries develop from the dorsal aorta relatively late in gestation, at about 8 weeks of gestation. When flow through the extrapulmonary arteries is abnormally diminished in late fetal life, or after birth, the bronchial arteries enlarge to supply the peripheral pulmonary arteries through connections between the pulmonary and bronchial circulations. Many such connections exist in the normal fetus and newborn, suggesting great adaptability, but they appear to decrease rapidly in size and number after birth. The structure of the pulmonary trunk reflects the high pulmonary vascular resistance of fetal life. It has a similar mural structure to that of the aorta, having concentric, compact parallel elastic fibres of uniform thickness.
Growth and Innervation after Birth
As the intrapulmonary arteries increase in size, their walls thicken to maintain a constant low relationship to the external diameter. In the arteries of the respiratory unit, smooth muscle cells differentiate from precursor intermediate cells and pericytes already in position, and as judged by light microscopy, non-muscular arteries become partially or completely muscularised. Muscle is said to have extended along the arterial pathway. The elastic laminas in the pulmonary trunk gradually become fragmented, and lose their ordered appearance. The wall of the pulmonary trunk becomes thinner relative to that of the aorta, with a ratio decreasing from unity to a mean value of 0.6 at about 8 months of age. 5 This ratio is maintained throughout life. The pulmonary vasculature is densely innervated at birth, and becomes more densely innervated with age. 6 In babies, as in adults, the nerves are predominantly of the sympathetic type. In pulmonary hypertensive infants, the abnormally thick-walled blood vessels are prematurely innervated by 3 months of age. 6
Regulation of Pulmonary Vascular Development
The presence of a circulation through the pulmonary vasculature from early embryonic and fetal life means that the circulation is exposed to external, distal influences from an early stage in its development. Circulating cells could contribute to building the vessel walls. Angioblasts can stream to developing organ beds from a considerable distance, 7 and incorporation of marrow-derived stem cells seems likely. The extent to which such cells retain their genetic memory, and the extent to which they can be modified by environmental factors, is of fundamental importance.
The innermost medial smooth muscle cells originate by migrating from bronchial smooth muscle. They have a migratory phenotype in the setting of obstructive pulmonary vascular disease. With respect to cytoskeletal composition, regulation of the actin cytoskeleton by RhoGTPases, and contractility, the smooth muscle cells from the inner and outer medial layers retain stable phenotypic differences when cultured. 8,9
These and other observations suggest that the cells may have been recruited into the vessel walls from afar. Because of this, they retain distinct hereditable characteristics which would enhance the heterogeneity of the vessel wall, and its potential for adaptation to environmental change. 10 The factors which determine whether an endothelial cell lying in the mesenchyme will become dedicated to either an arterial or a venous system are not understood. The tyrosine receptor EphB4, and its cognate ligand ephrin B2, discriminate between arteries and veins in the mouse, but less so in humans. Early angioblasts commit to either an arterial or a venous fate directed by a Notch gridlock signalling pathway. 11 It is possible, however, that pulmonary endothelial cells do not become committed until they have coalesced with an adjacent pulmonary artery or vein. It is at that time that the direction of flow, pressure, and circulating factors might influence commitment.
Experimental and gene targeting studies continue to emphasise the critical role of growth factors in lung development. Each appears to have a precise spatial and temporal expression pattern. In the human lung, we found eNOS, VEGF, and the VEGF receptors Flk-1and Flt-1and Tie-2, expressed in the capillary plexus at 38 days. 3 eNOS stimulates endothelial proliferation, migration, and formation of tubes, is a downstream mediator of growth factor–induced angiogenesis, and inhibits apoptosis. It is expressed on the endothelium of human arteries, capillaries, and veins throughout development. VEGF mediates vasculogenesis and angiogenesis. VEGF-A is required for vascular development throughout the embryo, and blood vessels do not form in mice deficient in the VEGF receptor Flk-1. 12,13 In cultured mouse lungs, VEGF is found at the branching points of peripheral airways. Grafting of beads coated with VEGF increases the density of the capillary bed. 14
The tyrosine receptor kinase Tie-2, and its ligand angiopoietin, is essential in formation of the capillary networks. 15 Also, endothelial phosphorylation of the Tie-2 receptor by angiopoietin leads to proliferation of smooth muscle cells, perhaps indicating that it has a role in the neomuscularisation of newly formed pulmonary arteries. 16,17 EGFmRNA and TGFαmRNA are expressed in the mesenchymal cells surrounding airways and alveoluses in the human fetal lung from 12 to 33 weeks of gestation, 18 but the receptors for these ligands were found in the airways and were involved in differentiation. Their direct role in vascular development is uncertain. Insulin-like growth factors I and II, and IGF-IR ligands and mRNA, become apparent at 4 weeks. 19 Anti-IGF-IR neutralising antibody reduced the number of endothelial cells and increased apoptosis of endothelial and mesenchymal cells in human lung explants. IGF-1 also upregulates VEGF, further emphasising the crucial role of this growth factor. A low oxygen tension, as occurs during fetal life, stimulates vascular and airway development. This involves HIF-1α, which upregulates expression of VEGF, and plays an important role in both vasculogenesis and angiogenesis. Studies on HIF-1α emphasise the importance of cross-talk between developing arteries and airways to ensure the normal development of both structures. 20
Functional Development in Fetal and Neonatal Life
The pulmonary endothelium plays a crucial role in adaptation to extra-uterine life. Its two most important functions are to help reduce pulmonary vascular resistance and to facilitate the clearance of alveolar fluid. 21
During fetal life, the endothelial cells are squat, have a narrow base on the subendothelium and a low ratio of surface to volume, with considerable overlap of lateral cell borders 22 ( Fig. 7-4 ). The small arterial lumen offers a high resistance to flow. Pulmonary vascular resistance is probably kept high during fetal life due to a significant release of vasoconstrictor substances, including endothelin-1 and leucotrienes, and low basal release of vasodilators such as nitric oxide and prostaglandin I 2 in the presence of a low oxygen tension. A mechanically induced increase in pulmonary blood flow, or exposure to vasodilators such as a raised oxygen tension, prostaglandin I 2 , and acetylcholine induces only transient vasodilation. 23

Endothelial Permeability
During fetal life, the lung is filled with fluid produced by the alveolar epithelium. 24 The liquid is absorbed by the alveolar epithelium at birth. Fetal pulmonary endothelial intercellular junctions are complex and fenestrated, while being tighter and less complex in older babies, indicative of improved barrier function. The higher endothelial permeability in fetal pulmonary vessels is probably due to the combined actions of hypoxia and a high level of circulating endothelin-1, VEGF, and angiotensin II. Endothelin-1 induces endothelial permeability, and its receptor antagonists can prevent capillary leakage. 25,26
VEGF, originally identified as a vascular permeability factor, is a potent inducer of plasma extravasation. It is produced in large quantities during fetal development. Angiotensin II may affect endothelial permeability via the release of prostaglandins and VEGF. 27 By contrast, an increase in nitric oxide has been shown to prevent endothelial leakage in the lung. The postnatal increase in nitric oxide, and the simultaneous reduction in endothelin, may contribute to tightening of endothelial junctions after birth. 28 Rho GTPases play an important role in maintaining endothelial junctional integrity, emphasising the necessity of sustaining a high ratio of Rac1 to RhoA. 29
The scaffolding proteins in the endothelial cell membrane may also change at birth, and contribute to the perinatal changes in endothelial barrier function. PECAM-1, also known as CD31, influences transendothelial migration of inflammatory cells, mechanosignal transduction, and angiogenesis. 30 Considerable amounts of this agent are expressed on fetal rat endothelial junctions, and expression decreases after birth when the barrier is formed between blood and gases. Caveolin-1 is a component of caveolas, an endothelial scaffolding protein which regulates the assembly of different signalling molecules at the plasma membrane. 31 Studies on doubly homozygous mice with caveolin-1 knocked out indicates that the protein plays a dual regulatory role in controlling lung microvascular permeability, acting as a structural protein required for formation of caveolas and caveolar transcytosis, and as a tonic inhibitor of eNOS negatively to regulate paracellular permeability. 32
Ensuring the Postnatal Fall in Pulmonary Vascular Resistance
Immediately after birth, the pulmonary arterial smooth muscle undergoes remodelling of the cytoskeletal actin, changing shape and showing an increase in the ratio of surface to volume ratio so as to achieve an increase in luminal diameter. 33 There is a transient reduction in the content of actin, and disassembly of actin filaments, with an increase in monomeric G-actin. The smooth muscular phenotype is regulated by the transcription factor serum response, which is itself negatively regulated by YY1. 34 The brief postnatal reduction in filamentous actin causes YY1 to relocate from cytoplasm to nucleus, thereby inhibiting the expression of differentiation markers, and increasing the activity of cell cycle genes. These changes do not occur in the pulmonary hypertensive state.
The transition to breathing air stimulates several physiological actions, including drainage of fetal lung fluid, rhythmic distension of the lung, and an increase in oxygen tension, all of which contribute to the fall in pulmonary vascular resistance. 35,36 The pioneering studies of Dawes and colleagues in Oxford in the 1960s on fetal sheep showed that mechanical ventilation reduced pulmonary vascular resistance, and that the response was enhanced when the inspired gas was enriched with oxygen. 37 Forty years later, no single factor has yet been identified as being primarily responsible for the initiation of vasodilation at birth. Nor do we know whether the endothelial cell, or the smooth muscle cell, is the prime target. It is probable that the abrupt expansion of the lungs leads to a cascade of events that facilitate the activation of vasodilatory responses, and reduce vasoconstrictor stimuli from the endothelium. The onset of breathing is associated with an increase in circulating bradykinin, and the release of prostaglandin I 2 and nitric oxide. 38,39 The process is thought to be caused by the sudden increase in flow imposing a sheer stress on the endothelium to promote their release. Although the pathway involving nitric oxide appears to play a crucial role in regulating the vasoreactivity of the transitional circulation, it is not essential. Mice deficient in eNOS survive, and there is no evidence that either iNOS or nNOS compensates for the absence of eNOS. 40 Irrespective of the signalling pathway under consideration, it is generally assumed that the pulmonary arteries are solely responsible for the postnatal fall in resistance. This is unlikely, since the pulmonary veins are not passive conduits. Newborn porcine pulmonary veins respond to acetylcholine to a greater extent than do pulmonary arteries. 41
Endothelial heterogeneity is evident even before birth. During embryonic development, the endothelial cells show high plasticity, and undergo constant changes in their expression of proteins to match the requirements of the developing vessel. 42,43 Heterogeneity is demonstrated in the newborn pulmonary circulation by the relaxant response to bradykinin, in which dependence on prostaglandin, nitric oxide, and a hyperpolarising factor differs in conduit and resistance arteries, and changes with age in each segment. 44,45 The regulation of these alternative signal transduction pathways in the immature normal and hypertensive pulmonary vasculature has still to be determined. Their tolerance to injury differs.
Role of Specific Mediators
Nitric Oxide
All three isoforms of nitric oxide synthase have been identified in the fetal lung. 46 Production of nitric oxide is regulated by transcription, post-transcriptional modification, availability of substrate, intracellular localisation, production of superoxides, and availability of co-factors. 47 Endothelial release is influenced by a variety of factors, including shear stress and tension of oxygen, which change dramatically at birth, and growth factors. The basal release of nitric oxide helps control resistance in the ovine fetal and transitional circulation. 48 Newborn isolated ovine and porcine conduit arteries and fetal and newborn porcine resistance arteries fail to relax to acetylcholine, the response maturing during the first 2 weeks of life. 23,44,49,50 By contrast, isolated fetal and newborn porcine pulmonary veins relax well in response to acetylcholine at birth, although like the arteries, the response improves with age. 41 Significant release of nitric oxide has also been demonstrated in newborn ovine pulmonary veins, possibly helping explain the experimental response of sheep to L-NAME, as noted above. A relatively poor receptor-mediated response is perhaps not surprising, given the marked changes occurring in the endothelial cell membrane as the ratio of surface to volume increases at birth. The density of muscarinic receptors increases rapidly immediately after birth, and the subtypes change. 51 But the relatively poor neonatal relaxant response is not restricted to receptor-dependant mechanisms, since the vessels do not relax in response to a calcium ionophore 49,50 Nor is the relatively poor endothelial-dependant relaxation at birth due to the pulmonary arterial smooth muscles cells being incapable of relaxation. The vessels relax in response to exogenous nitric oxide, although this response also improves significantly during the first 2 to 3 weeks of life. 49,50 There is no lack of nitric oxide synthase at birth. The expression of both the protein and the gene increases markedly towards term, and increases further to reach a maximum at 2 to 3 days of life. As in the adult, the predominant enzyme present at birth is the constitutive endothelial isoform.
There is no absolute or relative deficiency of the co-factor for nitric oxide synthase, BH4, at birth. 52 The efficacy of the enzyme, however, can be reduced by the action of endogenous inhibitors, primarily asymmetric dimethylarginine, which competes with the substrate l -arginine. 53 Levels of this inhibitor are high in amniotic fluid, increase towards term, and remain high in fetal blood. It would be expected to exert a significant tonic inhibitory effect on nitric oxide synthase at such concentrations. The inhibitor is certainly present in the urine of healthy newborn infants, and gradually declines to become undetectable by 5 days of age. 54 It is metabolised to citrulline by the dimethylarginine dimethylaminohydrolase enzymes, DDAH I and II, both of which are highly expressed in the fetal lung. Each isoform is developmentally regulated, and activity of the second increases rapidly immediately after birth. 55 Both asymmetric dimethylarginine and dimethylarginine dimethylaminohydrolase could play a significant role in the regulation of the fetal and newborn pulmonary vasculature.
The pulmonary arterial smooth muscle cell being targeted by the endothelium is itself changing rapidly. Basal accumulation of cGMP is high at birth, but falls rapidly to a lower adult level by 3 days of age. 50 This postnatal fall might be explained by the high expression of phosphodiesterase 5 (PDE5) and its hydrolytic activity found in the newborn rat lung. 56 Studies on lungs of several species also showed that PDE5 was responsible for a greater proportion of PDE activity during the first week of life than in the adult. 56,57 Despite the basal accumulation and enhanced accumulation of cyclic GMP in response to nitric oxide and its donors, the relaxation response of newborn vessels is less than might be expected. This might be accounted for by the smooth muscle cell membrane being more depolarised at birth than later. 58
Prostacyclin
Metabolism of arachidonic acid metabolism within endothelial cells leads to the production of vasoactive eicosanoids, either prostaglandins or leucotrienes. The circulating concentration of vasoconstrictor leucotrienes C 4 and D 4 decreases at birth and that of endothelial-derived prostacyclin increases, facilitating relaxation of the smooth muscle cells. The receptor for prostacyclin belongs to the family of G-protein coupled receptors. 59 Its effects are mediated by cAMP, though there may also be a cAMP-independent coupling of the receptor to the activation of potassium channels, which are important in the relaxation of smooth muscle cells. 60 The levels of prostacyclin during early fetal life are low, and this effect may be attributed to the inhibitory action of plasma glucocorticoids on endothelial transcription of the COX-1 gene, and expression of COX via a glucocorticoid receptor. 61 Levels increase at birth, a change coinciding with increasing levels of oestrogen in the fetal plasma. Physiologic levels of estradiol 17 activate synthesis of prostacyclin. 62 We lack information, however, about the function of the different subtypes of the prostacyclin receptor during development. Whether prostacyclin is crucial to the postnatal reduction in pulmonary arterial pressure is still uncertain. Expression of prostacyclin synthase is low at birth, and increases rapidly in the first week of life. Synthesis is higher in newborn than fetal vessels in the sheep, 63 as COX-1 activity is upregulated. The sudden increase in shear stress at birth, and the fall in pulmonary vascular resistance, coincide with the increased release of nitric oxide and prostacyclin, suggesting that the two agents may act in concert to effect the vasodilatory action of shear stress. Although inhibition of nitric oxide blocks the vasodilation resulting from increased oxygen and rhythmic pulmonary distension, COX inhibitors also attenuate vasodilation caused by rhythmic distension. 64
Endothelin
The level of circulating endothelin-1 is high in the normal fetus at term, and falls rapidly during the first week of life. Endothelial release of this potent vasoconstrictor is stimulated by hypoxia, the mechanical stimulation of shear stress and stretch, and chemical stimuli such as thrombin, norepinephrine, transforming growth factor-β, phorbol esters, and calcium ionophores. In the presence of high vascular tone, the agent has a vasodilatory effect, but similar infusions have a vasoconstrictor effect when pulmonary vascular tone is decreased during acute ventilation. It generally causes transient vasodilation followed by sustained vasoconstriction. It is abundant in the lung parenchyma and pulmonary arteries at birth, the expression decreasing initially, then increasing again, but at a lower level than in the newborn. Receptor binding sites are densely distributed over the smooth muscle cells of pulmonary vessels, with a relative increase in the B type with age. Between birth and 3 days, vasodilator receptors are transiently expressed on the pulmonary arterial endothelial cells. 65 This vasodilatory response to endothelin-1 increases after birth in both pulmonary arteries and veins. 66
Angiotensin
The role of the vasoconstrictor angiotensin in the regulation of the perinatal circulation is not understood. It is first expressed on the endothelium of large proximal pulmonary arteries in the pseudoglandular stage of lung development, and extends distally during gestation. 67 Expression of angiotensin-converting enzyme is 10 times lower at birth than in the mature rat lung. The vasoconstricting effects of angiotensin are largely due to angiotensin-mediated increases in the production of endothelin-1, 68 suggesting that its release may decrease immediately after birth.
Failure to Adapt to Extra-uterine Life: Persistent Pulmonary Hypertension of the Newborn
This condition has a high morbidity, and the rate of mortality remains at up to one-fifth despite the advent of treatment with inhaled nitric oxide. The pulmonary arteries fail to remodel after birth, and the vessels remain thick walled. The condition can be idiopathic but is more usually associated with hypoxia. It is a feature of certain types of congenital cardiac disease. Hence, it is multi-factorial in origin, but the nature of the underlying defect causing failure to adapt is uncertain. It has been shown to involve failure of endothelial-dependent and -independent relaxation, a primary structural abnormality of the targeted smooth muscle cells, and an excess of endothelin, possibly also with an excess of other vasoconstrictor agonists such as thromboxane or an isoprostane. Hypoxia, a common cause of the condition, alters the endothelial metabolism of vasoactive agents, such as endothelial nitric oxide synthase, 5-hydroxytryptamine, and angiotensin-converting enzymes. 69–71 It also increases endothelial permeability, which facilitates leakage of growth factors and blood cells into the underlying layer of smooth muscle cells. 29
Role of Specific Mediators
Nitric Oxide
Neonatal pulmonary hypertension affects both release of, and response to, nitric oxide in the pulmonary arteries. 41,50,72 Chronic hypoxia impairs endothelial calcium metabolism and normal coupling between the enzyme and caveolin-1, thus inactivating the enzyme. 69 Inappropriate postnatal persistence of an endogenous inhibitor of nitric oxide, such as ADMA, may also cause persistent pulmonary hypertension of the newborn, since it is known to persist at abnormally high levels after birth in such babies. 54 Arginine deficiency, absolute or relative, is a feature of the human infant with such persistent pulmonary hypertension, and an l -arginine infusion can be associated with an improvement in oxygenation.
Endothelin
The circulating levels of endothelin remain high in experimental models of hypoxia-induced persistent pulmonary hypertension. The density of binding of receptors, primarily the A type, is increased. 73 Co-constriction of pulmonary arteries and bronchuses is a well-recognised clinical feature of infants with pulmonary hypertension. Messenger RNA for preproendothelin was significantly elevated in fetal ovine lung tissue after ductal ligation during fetal life, and expression of the A type receptors was elevated. 74
Prostacyclin
The release of this agent is reduced in many forms of pulmonary arterial hypertension in humans, and is assumed to be low in newborns with persistent pulmonary hypertension. Its administration alleviates hypoxic pulmonary vasoconstriction in both afflicted newborn infants and experimental animals. Systemic hypotension can occur when this medication is used in sick infants.
Activation of Rho GTPases
Rho GTPases are key regulators of endothelial actin dynamics, thereby influencing both vascular reactivity and endothelial permeability. 21 Activation of RhoA has been associated with the development of pulmonary hypertension, 8,75 possibly by mediating the effects of vasoconstrictors such as angiotensin II, endothelin-1, and acetylcholine, and downregulating the expression of endothelial nitric oxide synthase in endothelial cells, and hence the production of nitric oxide. 21
Serotonin Fluoxetine
This selective inhibitor of the uptake of serotonin induced pulmonary hypertension in rats exposed to the drug during fetal life. Pulmonary arterial muscularity was increased. 76
Endothelial Permeability in Pulmonary Hypertension
Endothelial leakage occurs in pulmonary hypertension, irrespective of aetiology. Inhalation of nitric oxide may prevent pulmonary oedema, by both improving the barrier function of the endothelium and enhancing vascular relaxation. 77 Overexpression of angiotensin-1 produces leakage-resistant vessels, and angiotensin-1 is protective when used experimentally. 78 Potential therapeutic approaches include inhibition of VEGF, RhoA, and other molecules. 79 The natural mediator sphingosine 1-phosphate activates Rac1, and has considerable therapeutic potential. 80
Treatment
The aim is to control excessive reactivity and structural remodelling by using therapeutic agents which exploit the interactions between the signalling pathways controlling these events. Clinically, we rely on nitric oxide, inhibitors of phosphodiesterase 5, and less commonly, antagonists of the receptors to endothelin-1. Studies with guanylate cyclase activators are promising. Looking ahead, we know that in animal models the overexpression of vasodilator genes such as endothelial nitric oxide synthase, prepro-calcitonin gene-related peptide, and prostacyclin synthase, have produced promising results, 81 as has fasudil, the inhibitor of Rho kinase. 75 Statins might also be helpful, since they inhibit the prenylation and membrane translocation of Rho proteins, have anti-leakage and anti-inflammatory effects, and increase the availability of endothelial nitric oxide. Perhaps the most logical therapy would be an agent to open the potassium channels.
In summary, we are at last beginning to understand some of the mechanisms regulating pulmonary vascular development and function. Integrating clinical observations with experimental studies, taking advantage of gene targeting, and designing appropriate models of lung development present exciting challenges for the future.
PULMONARY CIRCULATION IN THE SETTING OF CONGENITALLY MALFORMED HEARTS
In children with congenitally malformed hearts, the pulmonary circulation may fail to develop normally, even before birth.
Abnormal Prenatal Development
Abnormalities of pulmonary vascular development arising during fetal life may be divided into primary developmental abnormalities that arise early in gestation and those that are probably secondary to haemodynamic changes caused by the presence of congenital heart disease and develop late in fetal life. Primary abnormalities include an absent right or left pulmonary artery, failure of some or all of the intrapulmonary arteries to connect exclusively with the central intrapericardial pulmonary arteries, a reduction in the number of generations of intrapulmonary arteries, and stenoses of the pulmonary arteries. These abnormalities may occur in children with a normal heart, when they are usually associated with disordered development of the airways. A persistent systemic arterial supply is a feature of a sequestrated segment of malformed hypoplastic lung. A reduction in the pulmonary arterial branching pattern is seen in the hypoplastic lung of renal agenesis and dysplasia, congenital diaphragmatic hernia, and rhesus isoimmunisation. 82–84 Pulmonary arterial stenoses occur in the rubella syndrome, with or without an associated intracardiac abnormality.
Absence of One Pulmonary Artery
Absence of one of the branches of the pulmonary trunk can occur in the presence of a patent pulmonary trunk when the pulmonary artery at the hilum is connected to a vessel arising from the ascending aorta or the aortic arch. This is sometimes known as a distal ductal origin of a pulmonary artery. Occasionally, the hilar pulmonary artery is connected to the right ventricle or aorta by an atretic thread in the position of a pulmonary trunk or arterial duct. In some cases, it is impossible at thoracotomy or postmortem to identify any such structure that might once have perfused the lung. In some children, serial angiographic studies show that the abnormality was acquired postnatally, usually in association with closure of the ducts. The intrapericardial pulmonary artery is then more correctly described as being interrupted rather than absent. Patients with tetralogy of Fallot are particularly vulnerable to such an acquired change. When no large systemic artery anastomoses with the hilar pulmonary artery, the pulmonary arterial supply is derived from an expanded bronchial circulation. When the intrapericardial pulmonary artery is absent or interrupted, however, the pulmonary artery is usually patent at the hilum, although it is frequently small. Consequently, all such patients should be regarded as potential candidates for correction by insertion of a prosthesis between the pulmonary trunk or right ventricle and the hilar pulmonary artery.
Failure of All Intrapulmonary Arteries to Connect Exclusively with the Intrapericardial Pulmonary Arteries
In the majority of patients with tetralogy of Fallot and pulmonary atresia, the lungs are perfused by large arteries arising from the aorta, called major systemic-to-pulmonary collateral arteries ( Fig. 7-5 ). 85 Development of the airways is normal. The intrapericardial pulmonary arteries are usually present, although they may be extremely small, or even incompletely canalised. Systemic-to-pulmonary collateral arteries are usually easily distinguishable from the enlarged bronchial arteries seen after birth in several types of congenital disease characterised by a low flow of blood to the lungs. The distinction is, however, sometimes blurred. Occasionally, arteries as large as major collateral arteries appear to have a similar origin from the aorta as do bronchial arteries, and then connect with intrapulmonary arteries to supply the lung. If, as seems probable, pulmonary and bronchial arteries have a common origin from the embryonic pulmonary plexus, this is not surprising. In the presence of collateral arteries, the source of arterial supply to the lungs is frequently complex, and varies in different patients. The complexity of their connections with the intrapulmonary arteries, and with each other, therefore, is also not surprising. In children with tetralogy and pulmonary atresia, a collateral artery may connect with a pulmonary artery at the hilum, and so perfuse both lungs if the intrapericardial pulmonary arteries are patent (see Fig. 7-5 ). Alternatively, a collateral artery may anastomose end-to-end with a lobar or segmental pulmonary artery and have no connection with the intrapericardial pulmonary arteries at either the hilum or within the lung. More than one collateral artery may perfuse the same intrapulmonary artery at the hilum, which is then said to have a duplicate, or dual, blood supply. Collateral arteries that do not connect with an intrapericardial or hilar pulmonary artery run to the hilum of the lung and then accompany a lobar bronchus until they anastomose with an intrapulmonary artery. As a result, a vessel that has the basic structure of a muscular systemic artery becomes a vessel with the structure of an elastic pulmonary artery. 86 The pulmonary artery then branches with the airways in a normal manner to perfuse the capillary bed, as in the normal lung. Different bronchopulmonary segments within a lobe may be connected to several different vessels, some segments being connected to an intrapericardial pulmonary artery, and others connecting directly with collateral arteries. Several arteries may, therefore, accompany the main and lobar bronchuses. On angiography, the orderly appearance of one pulmonary artery accompanying each bronchus is lost. The only method of ensuring that the anatomy is understood in each patient is to account for the arterial supply of each bronchopulmonary segment, look for sources of a duplicate supply to each segment, and search for connections between different collateral arteries.

The collateral arteries commonly arise from the descending thoracic aorta behind the origin of the left main bronchus in the presence of a left aortic arch, and almost in the midline when the arch is on the right. They may also arise from beneath the aortic arch and, less commonly, from below the diaphragm above the coeliac axis. They frequently divide near their aortic origin. Collateral arteries are commonly stenosed where they anastomose with a hilar or intrapulmonary artery. In postnatal life, a degree of stenosis is desirable to reduce the systemic arterial pressure to a level more suited to the pulmonary circulation. Collateral arteries, however, may be severely stenosed either at their origin from the aorta or, more commonly, in the mid-portion of the vessel between aorta and lung, where the lumen may even be occluded by large mounds of proliferating intimal tissue ( Figs. 7-6 and 7-7 ). It is not uncommon for children to become increasingly cyanosed during the first months of life, probably as a result of progressive intimal proliferation in the collateral arteries. Occasionally, children are born with minute intrapericardial and intrapulmonary arteries, and small or occluded collateral arteries. Multiple systemic-to-pulmonary collateral arteries seen in infants are best regarded as precarious fetal connections. Within the lung, the development of the pre- and intra-acinar arteries before and after birth depends on the lumen diameter of the perfusing vessels.


Most infants with tetralogy and pulmonary atresia suffer from the effects of hypoperfusion. Occasionally, the collateral arteries have a more elastic mural structure, being large vessels that provide a good, or even excessive, blood supply to the lung. Such patients present later. Large collateral arteries expose the distal intrapulmonary arteries to a high pressure, which can lead to the development of pulmonary vascular obstructive disease. When the lungs are perfused by an arterial duct, all the intrapulmonary arteries are usually connected to the hilum. A duct may, however, connect with a hilar pulmonary artery to perfuse some bronchopulmonary segments, while major systemic-to-pulmonary collateral arteries provide the only blood supply to other segments. Supply through bilaterally persistent arterial ducts can also be found.
Approximately two-thirds of patients present in infancy; at presentation, half are severely cyanosed and one-quarter are in heart failure. 87 In hypoperfused patients, the aim of surgery is to increase the supply to encourage growth of both intrapericardial and peripheral pulmonary arteries. All, or at least most, bronchopulmonary segments in each lung should connect, or be made to connect by unifocalisation, to an intrapericardial pulmonary artery or conduit, either as a prelude to, or at the time of, definitive repair. Collaterals should not be ligated unless an alternative source of arterial supply can be demonstrated. Since the anatomy is exceedingly variable, management must be individually tailored to each patient. The anatomy should be defined at presentation and the long-term management planned accordingly. Staged surgical procedures may be required to make a patient a candidate for complete repair. The morphological substrate is the limiting factor, and some patients will do better for longer without surgical intervention and eventually be candidates for transplantation.
Developmental Abnormalities of the Intrapulmonary Arteries
The various types of congenital cardiac disease may be regarded as vicarious experiments in which to study the relationship between changes in pulmonary arterial pressure and flow and structural development. Aortic valvar atresia, or critical stenosis, and pulmonary atresia with an intact ventricular septum, cause different and almost diametrically opposite structural abnormalities of the intra-acinar pulmonary arteries. In critical aortic stenosis or atresia, the entire cardiac output is ejected into the pulmonary trunk, and the body is perfused by the arterial duct. During fetal life, there is an increase in pulmonary arterial muscularity, and the number of intra-acinar arteries per unit area of lung may be increased. 88 At birth, the babies have severe pulmonary hypertension. It is now well established that those undergoing the Norwood sequence of operations can subsequently be converted to the Fontan circulation, indicating a marked reduction in pulmonary vascular resistance. By contrast, children with pulmonary atresia in whom the arterial duct is the only source of blood supply to the lung are born with abnormally small thin-walled pre- and intra-acinar arteries, and may have a reduction in the number of intra-acinar arteries. 89 Before birth, the pressure generated by the left ventricle is probably not transmitted to the pulmonary circulation across the rather small, abnormally angulated duct found in these patients. When the duct is relatively large, however, pulmonary arterial muscularity develops normally and intra-acinar arterial multiplication is almost normal.
Babies with totally anomalous pulmonary venous connection who present with obstructed venous return during the first week of life may show a marked increase in muscularity of both intra-pulmonary arteries and veins. 90,91 The findings are consistent with prenatal obstruction to pulmonary venous return. In those with the infradiaphragmatic type of anomaly, the descending vein can be narrowed or even occluded by fibrous tissue at birth. Also, the extrapulmonary veins may be smaller than normal and show intimal proliferation. Within the lungs, the increased muscularity is potentially reversible. In the extrapulmonary veins, however, prenatal obstruction may instigate intimal proliferation and fibrosis that can progress despite a technically successful operation. Post-operative stenosis then develops at some distance from the suture lines. Patients with the infradiaphragmatic type of anomaly are particularly vulnerable.
These findings in the pulmonary circulation of patients with aortic atresia or stenosis, pulmonary atresia, and totally anomalous pulmonary venous connection demonstrate the vulnerability of the fetal pulmonary circulation to changes in pressure and flow, and show how the structural changes in the lung can prejudice survival.
Effects of Pulmonary Hypoperfusion
The effects of hypoperfusion on the developing pulmonary circulation may be as deleterious as those of hyperperfusion. The intrapericardial pulmonary arteries may be minute. The proximal elastic arteries may contain less elastin than normal, while the intra-acinar arteries are usually smaller, and have a thinner muscle coat, than normal. Pulmonary arterial thrombosis can occur, leading to eccentric areas of intimal fibrosis, characterised by a peculiar form of recanalisation in which strands of fibrous tissue of varying thickness divide the arterial lumen into several channels. The aetiology and incidence of thrombotic lesions is uncertain, but they are thought to relate to the degree of polycythaemia. Enlargement of the bronchial arterial circulation to supplement, or even to replace, a normal pulmonary arterial blood supply occurs in many forms of cyanotic congenital heart disease, such as tetralogy of Fallot and pulmonary atresia. An enlarged bronchial arterial circulation is rarely found in newborn infants with an abnormally low pulmonary blood flow. Infants with pulmonary atresia and intact ventricular septum die when the duct closes because the bronchial arterial circulation is not sufficiently enlarged at that time. In older patients with tetralogy and pulmonary atresia, an enlarged bronchial arterial circulation is frequently seen at angiography. Serial cardiac catheterisation studies in such patients frequently show progressive enlargement of the bronchial arterial circulation. When the lung is severely hypoperfused, the development of the alveoluses can be impaired. Postmortem lung volume is reduced in children dying of tetralogy of Fallot. Performing a corrective operation before the end of the critical period of growth of the lung presumably encourages normal alveolar development. In addition, an early repair permits greater physical activity. In normal children, physical training increases total lung volume and vital capacity. Fortunately, the full impact of pulmonary hypoperfusion on the developing lung is rarely seen nowadays, since the flow can be increased as early as is necessary by medical or surgical treatment, be it palliative or corrective.
Growth of small pulmonary arteries is encouraged by surgically increasing the flow of blood through them for some time before performing the definitive repair. Whether the arteries will grow sufficiently to permit definitive repair depends upon their initial size, surgical expertise in increasing flow through them, and the extent of peripheral run-off. The potential for growth of both intrapericardial and peripheral pulmonary arteries is uncertain. Increased distensibility of a vessel consequent upon an increase in flow must be distinguished from growth. While in the reported studies the magnitude of the response and the relatively long time interval between operation and restudy suggests that growth has indeed occurred, we do not know whether or not these vessels will grow sufficiently to achieve a size appropriate to the size of the lung and that of the child. That the growth potential may not be normal is suggested by the abnormal structure of the wall of extrapulmonary vessels when pulmonary blood flow is low, abnormalities which change the mechanical properties of the wall and, hence, their physical response to an increase in flow.
PULMONARY HYPERTENSION
Pulmonary hypertension is a condition which causes immense suffering. A modest elevation in pulmonary arterial pressure is tolerable, but a high pressure is associated with obstructive pulmonary vascular disease leading to right-sided failure and death. The scene has changed so dramatically since the millennium. Improved diagnostic techniques facilitate assessment, and we now have a clinically useful classification ( Table 7-1 ). Pulmonary arterial hypertension is subdivided into the idiopathic and familial form and the pulmonary hypertension associated with other disorders, such as congenital cardiac disease. For those with established pulmonary vascular disease, discoveries of therapeutic agents have revolutionised management. They do not, however, cure the basic problem of pulmonary vascular disease.
|
|
|
|
|
Pulmonary hypertension itself is defined as a mean pressure within the pulmonary arteries equal to or greater than 25 mm Hg at rest, or 30 mm Hg on exercise, a definition which applies to all but the youngest infants.
The commonest causes of pulmonary hypertension in children are congenital heart disease and persistent pulmonary hypertension of the newborn. Children can also suffer from idiopathic pulmonary arterial hypertension, previously known as primary pulmonary hypertension. The patient is said to be suffering from the idiopathic form when there is no identifiable explanation for the increase in pulmonary arterial pressure. Idiopathic pulmonary arterial hypertension has been the subject of intense research because it is the most pure form of pulmonary hypertension, and new medicines have been evaluated and studied in this condition.
Pulmonary Hypertension in Those with Congenitally Malformed Hearts
Diagnosis of unrepaired congenital cardiac malformations calls for experienced assessment of likely operative outcomes, particularly the risk of acute post-operative and of long-term sustained pulmonary hypertension with continued evolution of pulmonary vascular disease.
Pre-operative Assessment of the Hypertensive Pulmonary Circulation
The cardiologist is constantly trying to predict the structural changes present in the lungs from the clinical, radiological, and haemodynamic findings without having to resort to a biopsy of the lung. Prediction can be difficult.
The clinical history is important. Cardiac failure may appear to improve as pulmonary vascular resistance increases. High flow of blood to the lungs is frequently associated with recurrent infections of the respiratory tract. Dilated pulmonary arteries compress the main and lobar bronchuses in children with and without pulmonary hypertension, leading to the familiar problem of recurrent collapse of different lobes or segments of lung. Not infrequently, the respiratory complications of congenital cardiac disease constitute anindication for corrective surgery in infancy. After operation, the bronchial deformity may persist for some time. Indeed, in some patients, prolonged compression appears to be associated with bronchomalacia. Rarely, babies may present to the paediatrician with a history of episodic collapse, indicative of pulmonary hypertensive crises. A labile pulmonary vasculature in the presence of an intact ventricular septum can be hazardous before as well as after surgical intervention. The extent of the problem is unknown.
The chest radiograph is reassuringly plethoric when the resistance is sufficiently low to permit a high flow to the lungs, and it is depressingly evident when pulmonary vascular disease is advanced. The development of severe obstructive disease in the muscular pulmonary arteries leads to peripheral pruning and a hypertranslucent appearance in association with dilation of the hilar and proximal vessels. Wedge angiography is not widely used, but it is helpful in demonstrating advanced pulmonary vascular obstructive disease, and should always be used in concert with other examinations in assessing whether the patient may still be able to undergo corrective surgery. If performed by balloon occlusion, the abnormal pulmonary wedge angiogram is characterised by decreased arborisation, reduced background opacification, and delayed venous filling. The arteries may appear tortuous, have segments of dilation or constriction, and have marginal defects suggestive of obliterative disease. Quantitative wedge angiography represents an attempt to improve the correlation between structure and function. 92 In the normal lung, the pulmonary arteries taper towards the periphery, but the vessels narrow over a shorter distance in the presence of an elevated pulmonary vascular resistance.
Pulmonary arterial pressure can be estimated non-invasively by Doppler ultrasound, but resistance cannot. At cardiac catheterisation, the lowest determinations of pulmonary arterial pressure and vascular resistance are conventionally accepted as a guide to outcome. This is based on the premise that, if the predominant structural abnormality in the pulmonary circulation is an increase in muscularity, it will respond to vasodilator substances. To this end, the patient is usually given nitric oxide. With any vasodilator, the release of vasoconstrictor tone will lower pulmonary vascular resistance and increase the magnitude of the left-to-right shunt, and may lower the pulmonary arterial pressure. Failure to achieve this response implies fixed and organic obstruction of the pulmonary circulation. Children with Down syndrome are particularly difficult to assess because they often suffer from obstruction of the upper airways, which may contribute to the increased pulmonary vascular resistance determined at cardiac catheterisation. The pathologist will then find less pulmonary vascular damage in a lung biopsy than expected for the increase in pulmonary arterial pressure and resistance. The use of oxygen to calculate vasoreactivity is usually more problematic than the use of nitric oxide. In both cases, consumption of oxygen should be measured, and dissolved oxygen should be taken into account when oxygen is added to nitric oxide to test reactivity.
The structural abnormalities present in the lung can usually be predicted from the type of intracardiac abnormality, the age of the child, and the haemodynamic findings at cardiac catheterisation. In more complex cardiac abnormalities, and if there is ever doubt about the likely outcome of intracardiac repair, an open lung biopsy should help clarify the position. It is also helpful in predicting prognosis even if the decision is taken to go ahead with the corrective operation.
Development of Pulmonary Vascular Disease
Children are occasionally born with pulmonary vascular disease, but this is rare. Pulmonary vascular disease usually starts at birth with abnormal postnatal remodelling, abnormal muscularisation of distal pulmonary arteries, and a reduction in the number of vessels. This is followed by obstructive intimal proliferation, and ultimately to formation of plexiform lesions if the pulmonary arterial pressure is allowed to remain high ( Fig. 7-8 ). Current thinking is that endothelial injury leads to programmed cell death and loss of small vessels. Endothelial injury is manifest as impaired endothelial dependent relaxation, defective levels of von Willebrand factor 93 and fibrinolysis. The progressive thickening of the wall of the more proximal intra-acinar and pre-acinar muscular arteries, and the obliteration associated with neointimal formation, has been related to heightened proliferation and migration of cells that have markers of smooth muscle cells because they are positive for α-smooth muscle actin. These cells may represent a specialised subpopulation, have originated as stem cells or fibrocytes, may be transformed fibroblasts or endothelial cells, or all of the above. With progressive obstruction, plexiform lesions develop. Endothelial proliferation is characteristic of this lesion, leading to the formation of aberrant channels in the obliterated lumen and in the adventitia. These lesions are thought to reflect clonal expansion of an endothelial cell resistant to death, 94 or of circulating endothelial progenitor cells. Pulmonary arterial endothelial cells from patients with idiopathic pulmonary hypertension are known to produce decreased amounts of nitric oxide on the basis of high levels of arginase, show exaggerated proliferation in response to growth factors, and exhibit high rates of glycolysis. 95 Additional pathological features include thickening of the pulmonary adventitial layers and venous hypertrophy. Immunohistochemical studies have revealed increased expression of transforming growth factor beta (TGF-β), matrix proteins such as collagen, elastin, fibronectin, tenascin-C, and glycosaminoglycans, macrophages, and T cells, as well as agents associated with inflammation, such as S100A4/Mts1 and fractalkine. 96
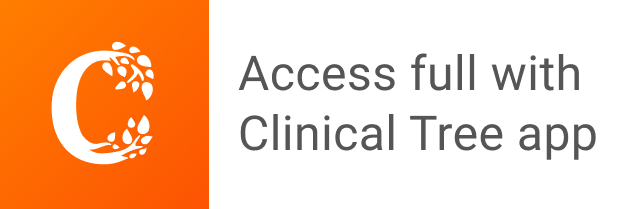