1 Pulmonary and Chest Wall Physiology Abstract An understanding of normal and abnormal pulmonary and chest wall physiology is important for those involved in thoracic surgery. Thoracic surgery often, but not always, involves patients with significant skeletal deformities, which in turn alters the structure of the thoracic cage and influences pulmonary function. Pulmonary function tests can be useful for formally assessing baseline pulmonary lung volumes and understanding existing disease states prior to surgery, as well as in postoperative assessment of pulmonary function. Here we review scoliosis, hyperkyphosis, and ankylosing spondylitis as they relate to baseline pulmonary changes, and changes that can occur following thoracic spine surgery. Restrictive and obstructive pathologies, postoperative pulmonary function, and pulmonary complications of thoracic spine surgery in general are likewise reviewed. Keywords: pulmonary, chest wall, thoracic wall, thoracic cage, respiratory, obstructive lung disease, restrictive lung disease, pulmonary function tests, scoliosis, ankylosing spondylitis, kyphosis Clinical Pearls • In patients with unexplained dyspnea, or known pulmonary or chest wall disorders, pulmonary function tests can be useful for diagnostic and prognostic purposes surrounding thoracic surgery. • Scoliosis, age-related hyperkyphosis, and ankylosing spondylitis are all skeletal abnormalities that may be associated with significant baseline and postoperative pulmonary dysfunction. • Restrictive lung physiology is common in patients with significant thoracic deformities. • Preoperative assessment of restrictive and obstructive lung disease may be done through spirometry alone, or more complete lung volume measurements may be obtained through pulmonary function testing. • The most commonly reported pulmonary complications following spine surgery include pulmonary embolism, respiratory distress, pulmonary edema, pneumothorax, pneumonia, and atelectasis. Familiarity with normal thoracic wall and pulmonary physiology is important for understanding commonly encountered thoracic surgical issues, and for the prevention and management of associated complications. The thorax we define here as the upper portion of the trunk extending between the neck and abdomen, and includes both intrathoracic contents (heart, lungs, thymus, distal trachea, and esophagus) and the thoracic wall. The thoracic wall is a dynamic structure composed of skin, fascia, nerves, vessels, muscles, and skeletal tissue, and serves the dual function of protecting intrathoracic contents, as well as the mechanical function of respiration.1 The primary physiological function of the lungs is to make external oxygen available for transport throughout the body, and to remove carbon dioxide that results from tissue metabolism.2 The skeletal structure of the thoracic wall includes 12 pairs of ribs and costal cartilage, 12 thoracic vertebrae, and the sternum. The first seven of the rib pairs are vertebrocostal ribs (true ribs), attaching directly to the sternum through their own costal cartilage. Rib pairs eight through ten are vertebrochondral and are joined to the cartilage of the rib just superior to them, and have an indirect connection to the sternum. Rib pairs eleven and twelve are free floating ribs that end in the posterior abdominal musculature. Costal cartilage on anterior rib articulations provide an element of elasticity to the thoracic wall, and help prevent anterior pressure or trauma from fracturing the ribs or sternum.1 The 12 paired ribs collectively form a large portion of the thoracic cage structure, and contain, among other important structures, intercostal muscles that form secondary muscles of respiration. Intercostal muscle contraction leads to both superior lateral and anterior–posterior expansion of the thoracic cage. Additionally, during forced inspiration, the pectoralis major muscles may assist with expansion of the thoracic cavity, and likewise the scalene muscles that extended from the neck to the first and second ribs also function as accessory muscles to elevate these ribs during forced inspiration. These accessory muscles and intercostal muscles are secondary to the primary muscle of respiration: the diaphragm. During diaphragmatic contraction, the central portion of the diaphragm descends inferiorly, creating a negative intrathoracic pressure gradient that allows for lung expansion. During normal respiration, as well as during deep breathing, the thoracic cage moves anteriorly, superiorly, and laterally, collectively increasing intrathoracic volume. The anterior–posterior diameter of the thorax can be further increased by straightening the back. During passive expiration, the intercostal muscles and diaphragm relax, resulting in increased intrathoracic pressure, and lung tissue that was previously expanded and stretched then recoils, and collectively this results in expiration of air.1 The lungs sit within the thoracic cage covered by visceral pleural, and inflate and deflate in response to changes in volume of the semirigid thoracic cage in which they are suspended.2 There are upper and lower lung lobes bilaterally, and the right lung also contains a middle lobe, whereas the left lung contains a lingula. All lung lobes are covered by visceral pleura, which abuts against either other lung tissue (where lung fissures exist), or the inner lining of the thoracic wall which is the parietal pleura. In between the visceral and parietal pleural lining exists a potential space with a normally negative pressure gradient that assists with lung expansion as the thoracic wall expands. This potential space is the pleural space and can fill (often partially) with air, fluid, and other inflammatory mediators during different pathological states. In its normal state the pleural space is thin, contains a minimal amount of fluid, and allows lung tissue to slide easily along the chest wall during lung inflation and deflation. The amount of air inspired into the bilateral lungs ranges normally from 4 L/min at rest to up to 100 L/min during maximum exercise.2 Lung volume measurements are traditionally obtained through pulmonary function testing (PFT), and key PFT measurements to be familiar with include total lung capacity (TLC), inspiratory capacity (IC), expiratory reserve volume, residual volume (RV), forced vital capacity (FVC), forced expiratory volume in 1 second (FEV1), and maximal voluntary ventilation (MVV). These are listed in Table 1.1 Pulmonary function measurements
1.1 Normal Physiology
1.1.1 Thoracic Wall
1.1.2 Pulmonary Function
Table 1.1 along with some other common measurements obtained during PFT that can be helpful for understanding normal versus pathological states. Note that lung capacities are composed of two or more lung volumes. PFT measurements are used often when evaluating unexplained dyspnea, severity of known pulmonary disease states, and preoperatively prior to thoracic surgery to provide baseline measurements, especially when baseline pulmonary dysfunction is suspected. Adequate patient effort is important when interpreting results of PFTs, and can be assessed by examining flow-volume loops that graph expiratory and inspiratory airflow against volume. Spirometry measurements, which are a subcomponent of PFTs, examine VC, FVC, FEV1, and MVV, and can provide such flow-volume loop diagrams. Spirometry measurements are provided in actual liters of air measured, as well as given as percentages predicted of comparison population norms.
Total lung capacity (TLC) | Volume in the lungs at maximal inhalation, or the sum of VC and RV |
Tidal volume (TV) | Volume of air moved into or out of the lungs during quiet breathing |
Inspiratory capacity (IC) | Sum of IRV and TV |
Inspiratory reserve volume (IRV) | Maximal volume of air that can be inhaled from the end-inspiratory position |
Expiratory reserve volume (ERV) | Maximal volume of air that can be exhaled from the end-expiratory position |
Residual volume (RV) | Volume of air remaining in the lungs after maximal exhalation |
Vital capacity (VC) | Volume of air exhaled following maximal inhalation |
Inspiratory vital capacity (IVC) | Maximum volume of air inhaled from the point of maximum expiration |
Forced vital capacity (FVC) | Vital capacity from a maximally forced expiratory effort |
Forced expiratory volume in one second (FEV1) | Volume of air exhaled at the end of the first second of forced expiration |
Functional residual capacity (FRC) | Volume in the lungs at the end-expiratory position (at the end of a resting tidal breath) |
Maximal voluntary ventilation (MVV) | Volume of air expired in a specified period during repetitive maximal effort |
Diffusing capacity for carbon monoxide (DLCO) | Diffusing capacity of carbon monoxide from lung alveolar space into the blood, used as a proxy for assessing the extent to which oxygen passes from the alveolar space into the blood |
RV/TLC% | Residual volume expressed as a percent of TLC |
Peek expiratory flow (PEF) | Highest forced expiratory flow measured with a peak flow meter |
PFTs when abnormal can be broadly grouped into obstructive or restrictive processes, although many disease states involve elements of both. Reduction in TLC (< 80%) is indicative of restrictive lung disease. Such restriction can be external to the lung parenchyma, due to compression from severe obesity, scoliosis, or secondary to neuromuscular weakness. Restriction can alternatively be due to intrinsic lung pathology such as pulmonary fibrosis, among other disease states. Obstructive lung disease involves airflow obstruction (defined as FEV1/FVC ratio of < 70%), and includes common conditions such as asthma and chronic obstructive pulmonary disease (COPD). Asthma involves reversible airflow obstruction (i.e., there is some improvement noted following inhalation of a bronchodilator) versus COPD which is generally nonreversible. Although again, even these conditions can overlap, such as with asthma–COPD overlap syndrome, where there are components of reversibility and nonreversibility.
The diffusing capacity of the lungs for carbon monoxide (DLCO) measures gas exchange at the alveolar level across the alveolar–capillary membrane. Measurement of DLCO is done during PFTs and entails inhaling a very low concentration of carbon monoxide and subsequently measuring the amount of carbon monoxide exhaled to infer the amount that was exchanged at the alveolar–capillary membrane. Disease states resulting in low DLCO measurements include vasculitis, pulmonary embolism, pulmonary hypertension, pulmonary fibrosis, and emphysema in which there is decreased alveolar surface area, and restrictive lung disease in which there is decrease in total lung area. Carbon monoxide uptake within capillaries is dependent on the presence of hemoglobin, and thus disease states with low or abnormal hemoglobin may be associated with abnormally low DLCO measurements independent of pulmonary pathology.3
1.2 Abnormalities Associated with Common Spinal Pathologies
1.2.1 Scoliosis
Scoliosis is the most common spinal disorder which directly impacts the thoracic cage. It is generally thought to cause a restrictive pattern of lung dysfunction with decreased TLC. However, significant reductions in lung volume do not typically manifest until spinal deformity becomes more severe ( Table 1.2). Other factors, such as loss of thoracic kyphosis, cephalad curve location, vertebral rotation, and greater number of involved vertebrae also contribute to diminished TLC.4,5 These factors result in a loss of thoracic cage volume6,7 and a chest wall that is less compliant,8 thereby increasing the work of breathing. Pulmonary hypertension and chronic respiratory failure may develop in scoliosis patients with severe deformities.9 Pulmonary hypertension, especially when severe, is particularly poor prognostic factor for perioperative morbidity and mortality, and is an important factor to identify preoperatively. This can be done noninvasively via echocardiogram measurements, which provides an estimation, or performed invasively for more accurate measurements via right heart catheterization. In the case of early-onset scoliosis, the presence of thoracic deformity during the time of rapid lung development may result in true pulmonary hypoplasia, further compounding respiratory deficits and potentially resulting in thoracic insufficiency syndrome (an inability of the thorax to support normal respiration and lung growth).10,11
Table 1.2 Scoliosis severity and clinical manifestations
Cobb’s angle | Potential clinical findings |
< 10° | None |
> 25° | Possible pulmonary artery pressure increase on echocardiogram |
> 70° | Likely significant decreased pulmonary volume |
> 100° | Dyspnea with exertion |
> 120° | Likely alveolar hypoventilation, chronic respiratory failure |
Source: Data from Koumbourlis.12 |
The typical PFT profile of a patient with scoliosis is one of diminished TLC and IC with less of a deleterious effect on RV and functional residual capacity (FRC).13,14 As such, the RV:TLC ratio is frequently elevated. An elevated RV:TLC ratio is traditionally viewed as evidence of obstructive airway disease; however, in patients with scoliosis this is not necessarily the case, and an elevated ratio is usually more reflective of diminished TLC specifically. Airway resistance may be increased because of distortion caused by chest wall deformity and by chronic airway inflammation due to inadequate clearance of secretions.15,16 This predisposes patients, especially those with neuromuscular disease, to pneumonia.13 Ventilation/perfusion mismatch has also been shown to occur with scoliosis.17,18 This may explain the mild hypoxemia with normocapnia observed in many patients with scoliosis.9,14 With severe deformities, however, hypercapnia develops and may limit the response to carbon dioxide,4,19,20,21 resulting in sleep-disordered breathing.22
During exercise or stress, patients with significant scoliosis attempt to increase minute ventilation (the volume of gas inhaled or exhaled from the lungs per minute) via an elevated respiratory rate rather than an increased tidal volume. Work of breathing is further increased as the dead space must be exchanged with each shallow breath. This compensatory mechanism is particularly ineffective in patients with neuromuscular scoliosis as they are at an elevated risk of muscle fatigue and respiratory failure.13 It has also been observed that exercise capacity may even be diminished in patients with milder curves.23,24
1.2.2 Ankylosing Spondylitis
Ankylosing spondylitis (AS) is an inflammatory condition that typically affects the sacroiliac joints first, then moves rostral along the spine, and involves the chest wall later in the course of the disease.13,25 It produces eventual ankyloses of the spine and sternocostal joints as well as rib cartilage calcification.26 The spine assumes a position of rigid hyperkyphosis with the thoracic cage fixed in the inspiratory position.27 In addition to structural abnormalities, AS may directly affect the lungs, typically via interstitial disease.28 While it is unusual for parenchymal lung involvement to directly produce clinically significant changes in pulmonary function, it may predispose patients to infection and pneumothorax.29
PFT in the setting of AS reveals a restrictive pattern combined with hyperinflation. Vital capacity is diminished in proportion to the severity of disease, while FRC and RV are normal or elevated in the setting of air trapping.29 Increased diaphragmatic and abdominal wall movements compensate for diminished thoracic cage mobility.30,31,32,33,34 Small airway obstruction caused by interstitial lung disease may also be present.35,36,37 Arterial blood gasses and DLCO tend to be normal unless the lung involvement is severe.13 The literature is mixed on whether thoracic involvement in AS adversely effects exercise tolerance.37,38,39,40,41
1.2.3 Age-Related Hyperkyphosis
The saying “age is a kyphosing process” reflects the tendency of degenerative changes in the spine to produce increasing kyphosis over time.42 Mean thoracic kyphosis increases from approximately 25 degrees in people age 18 to 29, to 65 degrees in those aged 75 and older,43 with women more rapidly affected than men.44 Between 20 and 45% of older adults exhibit hyperkyphosis,45 typically defined as a thoracic kyphosis angle exceeding 40 degrees.44,46 Osteoporosis-related vertebral compression fractures often play a role in the development of kyphosis—each vertebral compression fracture contributes an average of 3.8 degrees toward overall thoracic kyphosis.47 Anterior intervertebral disc wedging from thoracic degenerative disc disease,47,48,49,50 loss of paraspinal muscle strength,51,52 and general deconditioning53
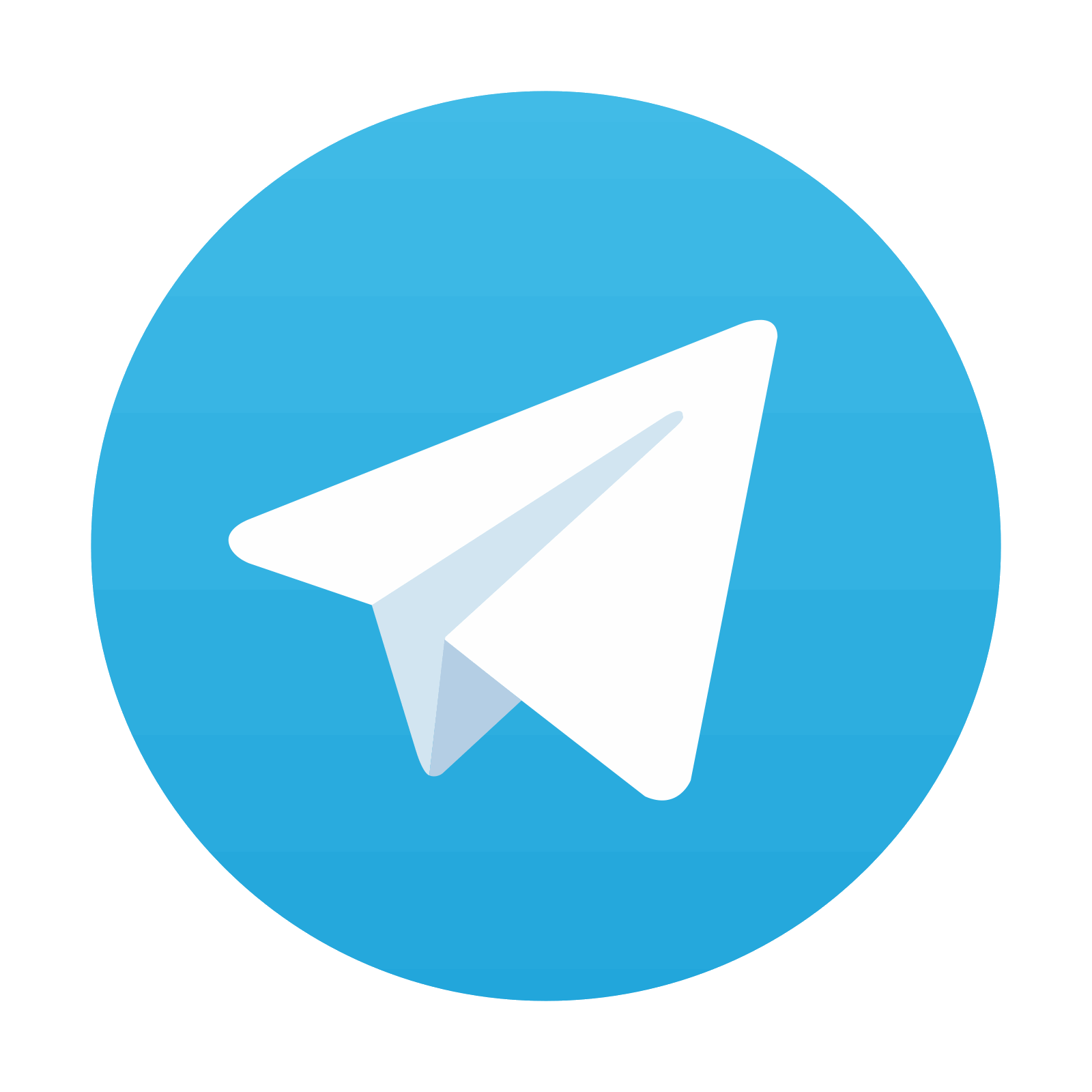
Stay updated, free articles. Join our Telegram channel
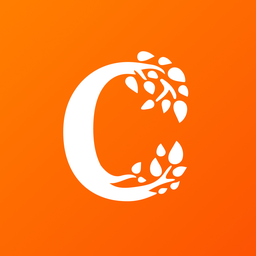
Full access? Get Clinical Tree
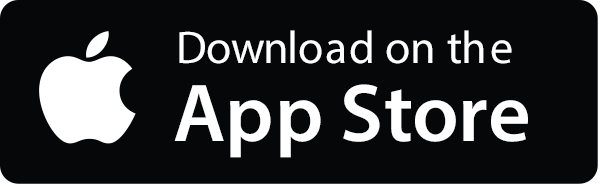
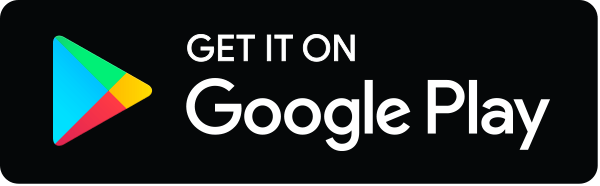