1 To be effective at gas exchange, the lungs cannot act in isolation. They must interact with the central nervous system (which provides the rhythmic drive to breathe), the diaphragm and muscular apparatus of the chest wall (which respond to signals from the central nervous system and act as a bellows for movement of air), and the circulatory system (which provides blood flow and thus gas transport between the tissues and lungs). The processes of oxygen uptake and carbon dioxide elimination by the lungs depend on proper functioning of all these systems, and a disturbance in any of them can result in clinically important abnormalities in gas transport and thus arterial blood gases. This chapter begins with an initial overview of pulmonary anatomy, followed by a discussion of mechanical properties of the lungs and chest wall, and a consideration of some aspects of the contribution of the lungs and the circulatory system to gas exchange. Additional discussion of pulmonary and circulatory physiology is presented in Chapters 4, 8, and 12; neural, muscular, and chest wall interactions with the lungs are discussed further in Chapter 17. It is appropriate when discussing the anatomy of the respiratory system to include the entire pathway for airflow from the mouth or nose down to the alveolar sacs. En route to the alveoli, gas flows through the oropharynx or nasopharynx, larynx, trachea, and finally a progressively arborizing system of bronchi and bronchioles (Fig. 1-1). The trachea divides at the carina into right and left mainstem bronchi, which branch into lobar bronchi (three on the right, two on the left), segmental bronchi, and an extensive system of subsegmental and smaller bronchi. These conducting airways divide approximately 15 to 20 times down to the level of terminal bronchioles, which are the smallest units that do not actually participate in gas exchange. Further details about the anatomy of airways, alveoli, and the pulmonary vasculature, particularly with regard to structure-function relationships and cellular anatomy, are given in Chapters 4, 8, and 12. Mechanical Aspects of the Lungs and Chest Wall Alternatively, instead of positive pressure exerted on alveoli through the airways, negative pressure could be applied outside the lungs to cause their expansion. Thus, what increases the volume of the isolated lungs from the resting, essentially airless, state is application of a positive transpulmonary pressure—the pressure inside the lungs relative to the pressure outside. Internal pressure can be made positive, or external pressure can be made negative; the net effect is the same. With the lungs inside the chest wall, the internal pressure is alveolar pressure, whereas external pressure is the pressure within the pleural space (Fig. 1-2). Therefore, transpulmonary pressure is defined as alveolar pressure (Palv) minus pleural pressure (Ppl). For air to be present in the lungs, pleural pressure must be relatively negative compared with alveolar pressure. The relationship between transpulmonary pressure and lung volume can be described for a range of transpulmonary pressures. The plot of this relationship is the compliance curve of the lung (Fig. 1-3, A). As transpulmonary pressure increases, lung volume naturally increases. However, the relationship is not linear but curvilinear. At relatively high volumes, the lungs reach their limit of distensibility, and even rather large increases in transpulmonary pressure do not result in significant increases in lung volume. The compliance curve of the chest wall relates the volume enclosed by the chest wall to the pressure across the chest wall (Fig. 1-3, B). The curve becomes relatively flat at low lung volumes at which the chest wall becomes stiff. Further changes in pressure across the chest wall cause little further decrement in volume. To examine how the lungs and chest wall behave in situ, remember that the elastic properties of each are acting in opposite directions. At the normal resting end-expiratory position of the respiratory system (functional residual capacity [FRC]), the lung is expanded to a volume greater than the resting volume it would have in isolation, whereas the chest wall is contracted to a volume smaller than it would have in isolation. However, at FRC the tendency of the lung to become smaller (the inward or elastic recoil of the lung) is exactly balanced by the tendency of the chest wall to expand (the outward recoil of the chest wall). The transpulmonary pressure at FRC is equal in magnitude to the pressure across the chest wall but acts in an opposite direction (Fig. 1-3, C). Therefore pleural pressure is negative, a consequence of the inward recoil of the lungs and the outward recoil of the chest wall. The chest wall and the lungs can be considered as a unit, the respiratory system. The respiratory system has its own compliance curve, which is essentially a combination of the individual compliance curves of the lungs and chest wall (see Fig. 1-3, C). The transrespiratory system pressure, again defined as internal pressure minus external pressure, is airway pressure minus atmospheric pressure. At a transrespiratory system pressure of 0, the respiratory system is at its normal resting end-expiratory position, and the volume within the lungs is FRC. At the other extreme, when we exhale as much as possible, we reach residual volume (RV). At this point a significant amount of gas still is present within the lungs—that is, we can never exhale enough to empty the lungs entirely of gas. Again, the reason can be seen by looking at the compliance curves in Figure 1-3, C. The chest wall becomes so stiff at low volumes that additional effort by the expiratory muscles is unable to decrease the volume any further. Therefore, RV is determined primarily by the balance of the outward recoil of the chest wall and the contracting action of the expiratory musculature. However, this simple model for RV applies only to the young individual with normal lungs and airways. With age or airway disease, further expulsion of gas during expiration is limited not only by the outward recoil of the chest wall but also by the tendency for airways to close during expiration and for gas to be trapped behind the closed airways. To maintain normal gas exchange to the tissues, an adequate volume of air must pass through the lungs for provision of O2 to and removal of CO2 from the blood. A normal person at rest typically breathes approximately 500 mL of air per breath at a frequency of 12 to 16 times per minute, resulting in a ventilation of 6 to 8 L/min (minute ventilation [ This defines the major factors determining PaCO2. When a normal individual exercises, For gas coming directly from alveoli that have participated in gas exchange, PCO2 approximates that of arterial blood. For gas coming from the dead space, PCO2 is 0 because the gas never came into contact with pulmonary capillary blood. West developed a model of pulmonary blood flow that divides the lung into zones, based on the relationships among pulmonary arterial, venous, and alveolar pressures (Fig. 1-4). As stated earlier, the vascular pressures—that is, pulmonary arterial and venous—depend in part on the vertical location of the vessels in the lung because of the hydrostatic effect. Apical vessels have much lower pressure than basilar vessels, the difference being the vertical distance between them (divided by a correction factor of 1.3 to convert from cm H2O to mm Hg).
Pulmonary Anatomy and Physiology
The Basics
Anatomy
Physiology
Ventilation
]).* The volume of each breath (tidal volume [VT]) is not used entirely for gas exchange; a portion stays in the conducting airways and does not reach the distal part of the lung capable of gas exchange. The portion of the tidal volume that is “wasted” (in the sense of gas exchange) is termed the volume of dead space (VD), and the volume that reaches the gas-exchanging portion of the lung is the alveolar volume (VA). The anatomic dead space, which includes the larynx, trachea, and bronchi down to the level of the terminal bronchioles, is approximately 150 mL in a normal person; thus, 30% of a tidal volume of 500 mL is wasted.
increases, but
increases proportionately so that PaCO2 remains relatively constant.
Circulation
Stay updated, free articles. Join our Telegram channel
Full access? Get Clinical Tree
Pulmonary Anatomy and Physiology: The Basics
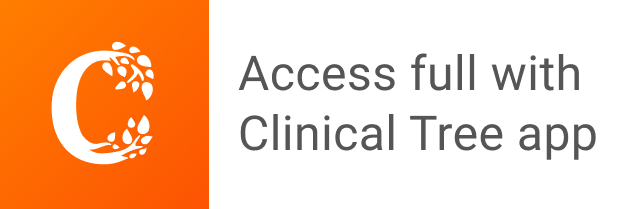