Principles of Mapping and Ablation
Yuliya Krokhaleva
Noel G. Boyle
Kalyanam Shivkumar
Eric Buch
INTRODUCTION
Catheter ablation and antiarrhythmic medications are the two modalities currently used for the treatment of cardiac arrhythmias. Although catheter ablation is first-line therapy for supraventricular tachycardia and typical atrial flutter due to high success rate and low incidence of complications, it is increasingly utilized as an early treatment strategy for complex more supraventricular and ventricular arrhythmias. Antiarrhythmic drugs have limited efficacy for many arrhythmias, potential for toxicity and interactions with other medications, and risk of proarrhythmia. These considerations have led to more widespread use of catheter ablation in patients with ventricular tachycardia (VT), atrial fibrillation, and atypical atrial flutter. Successful treatment of cardiac arrhythmias with catheter ablation requires correct identification of the rhythm and clear understanding of its mechanism. This is usually achieved by performing an invasive electrophysiology study (EPS). The EPS allows for precise determination of the arrhythmia mechanism and localization of the arrhythmogenic focus or reentry circuit. Typically, both the EPS and ablation are performed within the same procedure with the EPS done first and followed by the ablation procedure.
The purpose of this chapter is to discuss general principles of mapping and ablation for both supraventricular and ventricular arrhythmias. After a brief discussion of the historical development of catheter ablation and the biophysics of energy delivery, various mapping techniques and their application in the ablation of specific arrhythmias will be reviewed.
CATHETER ABLATION: HISTORICAL DEVELOPMENT
The first nonpharmacologic therapy for cardiac arrhythmias was surgical. In the late 1960s, the development of intraoperative epicardial mapping allowed effective treatment of Wolff-Parkinson-White syndrome by surgical disruption of accessory pathway fibers. As in catheter ablation procedures, the goal was to interrupt electrical conduction to
prevent recurrent arrhythmia. A successful ablation precisely targets critical tissue, creating permanent lesions while minimizing damage to surrounding structures.
prevent recurrent arrhythmia. A successful ablation precisely targets critical tissue, creating permanent lesions while minimizing damage to surrounding structures.
Building on the success of arrhythmia surgery and the development of transvenous recording of intracardiac signals using electrode catheters, Scheinman et al. performed the first catheter-based ablation in 1982. They used high-energy direct-current shocks to cause complete atrioventricular (AV) block for rate control in patients with atrial arrhythmias in combination with implantation of a permanent pacemaker. Energy was delivered between the tip of a catheter located near the His bundle and a grounding pad on the skin, creating a plasma arc and steam bubble that destroyed AV junctional tissue and caused permanent AV block. Though effective, the force of the energy discharge frequently resulted in damage to surrounding structures, and sometimes caused cardiac perforation or even death. In-hospital mortality rates approached 6%, which prevented widespread application of the technique.
The use of radiofrequency (RF) energy for catheter ablation was first reported for AV nodal ablation by Huang et al. in 1987. By 1991, RF energy had supplanted direct-current shock due to its improved safety profile. Adapted from surgical electrocautery, RF ablation uses low-voltage, high-frequency alternating current of 500 to 1,000 kHz, delivering energy between the catheter tip and a large dispersive grounding pad on the skin. RF energy is applied from the tip of the catheter to the endocardial or epicardial surface of the heart. This results in a controlled focal lesion in the targeted arrhythmogenic tissue, due to resistive heating and thermal conduction. Since lesion size depends on delivered power and tissue temperature, both of which can be adjusted by the operator, this method offers more precise control over the tissue being ablated. A wide variety of ablation catheters and guiding sheaths allow access to virtually any part of the heart. Due to the safety and efficacy of RF catheter ablation, it is the most common energy source for catheter ablation.
BIOPHYSICS OF CATHETER ABLATION
When a sustained tissue temperature of 50°C is achieved, the result is tissue desiccation and protein denaturation, leading to irreversible loss of electrical conduction, which is the goal of catheter ablation (Fig. 9-1). With temperature-controlled energy delivery, the RF generator automatically reduces power when a preset temperature is reached at the tip of the ablation catheter. This minimizes the risk of coagulum
formation and steam pops from excessive heating. Damage is generally limited to tissue within a few millimeters of the catheter tip, since heat generated by resistive heating decreases as the fourth power of distance from the catheter tip.
formation and steam pops from excessive heating. Damage is generally limited to tissue within a few millimeters of the catheter tip, since heat generated by resistive heating decreases as the fourth power of distance from the catheter tip.
During an ablation procedure, the operator can manipulate various parameters to control lesion size and depth, including catheter contact, power, duration of RF delivery, and maximum temperature. Newer technologies have been developed to create larger lesions and improve ablation efficacy, including catheters with larger tips (>4 mm), internally cooled catheters, and open-irrigated catheters that flush saline through holes at the tip of the catheter (Fig. 9-2). Internally cooled catheters permit the delivery of more power without excessive heating of the tissue-catheter interface, resulting in reliable energy delivery that creates deeper lesions with less coagulum formation. Open-irrigated catheters offer even greater tissue interface cooling and further reduce the incidence of thrombus formation and steam pops. However, with all of these technologies that increase lesion size and depth, the risk of collateral damage to surrounding structures rises.
OTHER ENERGY SOURCES FOR ABLATION
Although RF energy is used in the vast majority of ablation procedures, other energy sources are also available. In cryothermy, also called cryoablation, tissue is destroyed not by electrical heating, but rather by extreme cooling. The first transvenous catheter ablation using cryothermy was reported by Khairy et al. in 1999. The cryoablation catheter is cooled by injection of liquid nitrous oxide into the catheter, which cools as it changes to the gas phase, resulting in temperatures of -75°C. This damages tissue by creating extracellular ice crystals, drawing water out of cells. When the tissue thaws, hypotonic fluid flows back in, causing swelling and cell membrane rupture. A second mechanism of lesion formation from cryotherapy is vascular-mediated tissue injury.
When the intended ablation site is near the vital structure (e.g., midseptal and para-hisian accessory pathways, difficult cases of AV nodal reentrant tachycardia),
cryoablation provides some safety advantages. It offers the opportunity for reversible functional mapping by creating a “test lesion” at an intermediate low temperature of -30°C at the intended ablation site, thus avoiding permanent damage. If mild cooling has no effect at all or leads to temporary injury and loss of function of the vital structure, no further ablation is performed at that site given lack of efficacy or potential damage. The tissue is thawed with full restoration of function and catheter is repositioned. Once mild cooling is determined to be safe and has the desired effect, the “test lesion” can be followed by cooling the catheter tip to -75°C for 4 min, producing a permanent lesion. Another reported advantage of cryoablation is increased catheter stability due to adherence of the catheter tip to frozen tissue. This may be particularly advantageous when ablation target is a highly mobile structure such as papillary muscle containing a focus of VT. In addition, cryoablation results in creation of smaller, better demarcated lesions and less thrombus formation as compared to RF ablation. Disadvantages of cryoablation include lower acute efficacy and higher arrhythmia recurrence rate, likely due to incomplete tissue destruction.
cryoablation provides some safety advantages. It offers the opportunity for reversible functional mapping by creating a “test lesion” at an intermediate low temperature of -30°C at the intended ablation site, thus avoiding permanent damage. If mild cooling has no effect at all or leads to temporary injury and loss of function of the vital structure, no further ablation is performed at that site given lack of efficacy or potential damage. The tissue is thawed with full restoration of function and catheter is repositioned. Once mild cooling is determined to be safe and has the desired effect, the “test lesion” can be followed by cooling the catheter tip to -75°C for 4 min, producing a permanent lesion. Another reported advantage of cryoablation is increased catheter stability due to adherence of the catheter tip to frozen tissue. This may be particularly advantageous when ablation target is a highly mobile structure such as papillary muscle containing a focus of VT. In addition, cryoablation results in creation of smaller, better demarcated lesions and less thrombus formation as compared to RF ablation. Disadvantages of cryoablation include lower acute efficacy and higher arrhythmia recurrence rate, likely due to incomplete tissue destruction.
Cryotherapy with the cryoballoon catheter (Arctic Front Advance, Medtronic, Minneapolis, MN) is widely used for ablation of paroxysmal atrial fibrillation (Fig. 9-3). The cryoballoon delivers a circumferential lesion at the antrum of the pulmonary vein as opposed to point-by-point RF ablation. Usually, two to three 3-minute freezes per vein result in complete pulmonary vein isolation. Procedure time is reduced with cryoballoon ablation. However, rate of phrenic nerve injury is higher with cryoballoon compared to RF ablation for pulmonary vein isolation, because the entire balloon is cooled, and there is no way to selectively spare ablation of the anterior segments of the right-sided pulmonary veins.
Other methods of catheter-based energy delivery are currently in development. These include bipolar RF ablation, in which energy is delivered between two catheter
electrodes, rather than between an electrode and skin pad as in traditional unipolar RF. Novel non-RF energy sources are also being tested in animal and human studies. These include laser, microwave, and high-intensity focused ultrasound, some of which will be delivered through newly developed catheters designed to increase the safety and efficacy of ablation.
electrodes, rather than between an electrode and skin pad as in traditional unipolar RF. Novel non-RF energy sources are also being tested in animal and human studies. These include laser, microwave, and high-intensity focused ultrasound, some of which will be delivered through newly developed catheters designed to increase the safety and efficacy of ablation.
CATHETER MAPPING AND ABLATION: VASCULAR ACCESS AND CARDIAC CHAMBER APPROACH
To perform EPS and catheter ablation, venous access from multiple sites is typically required. Most commonly, bilateral femoral veins are cannulated using Seldinger technique. Often, the internal jugular vein is accessed as well, usually for placement of a catheter into coronary sinus. Multipolar catheters are advanced into cardiac chambers to record electrical activity. Generally, catheters are positioned in the high right atrium, bundle of His, right ventricle, and coronary sinus. The number of catheters used and their positions are dependent on the clinical question that needs to be answered. An ablation catheter is typically advanced via the right femoral vein. It can be utilized for both mapping and ablation.
Transvenous approach allows direct access to the right atrium through the inferior vena cava for ablation of right atrial flutter, right atrial tachycardia, right-sided accessory pathways, or AV nodal modification. The ablation catheter can also be advanced across the tricuspid valve into the right ventricle or right ventricular outflow tract for ablation of VT or premature ventricular complexes (PVCs) arising from these areas.
Ablation within the left atrium, for treatment of atrial fibrillation, left atrial tachycardia, left atrial flutter, or left-sided accessory pathways, is usually performed by the transseptal approach. In this procedure, a long guiding sheath is placed via the femoral vein into the right atrium, and a specialized needle is used to puncture the fossa ovalis for access to the left atrium, under fluoroscopic or echocardiographic (intracardiac or transesophageal) guidance (Fig. 9-4). After the needle is advanced across the fossa ovalis, it is of paramount importance to confirm that the needle is within the left atrium before advancing the dilator and the sheath. This can be done by recording left atrial pressure and observing distribution of contrast injection by ICE or fluoroscopy.
Access to the left ventricle, for ablation of VT or left ventricular PVCs, can be obtained by either the atrial transseptal or retrograde aortic approach. With the atrial transseptal approach, the catheter first enters the left atrium and it is then advanced across the mitral valve into the left ventricle. The transseptal approach is generally preferred as it provides a more favorable catheter profile for ablation of most areas within the left ventricle. With the retrograde aortic approach, the ablation catheter is advanced through the femoral artery to the ascending aorta and across the aortic valve.
It must be emphasized that any mapping or ablation procedure on the left side of the heart requires systemic anticoagulation, usually with intravenous unfractionated heparin, to prevent thrombus formation and thromboembolic complications, including stroke. This is done in all patients undergoing left-sided procedures, even those who are therapeutically anticoagulated with either warfarin (international normalized ratio 2-3) or novel anticoagulants at the time of the procedure. The left atrium is accessed by trans-septal catheterization, and the left ventricle can be accessed by a trans-septal or retrograde aortic route. The activated clotting time (ACT) should be monitored frequently to ensure adequate anticoagulation for the duration of left heart access.
Although all of the cardiac chambers can be accessed with intravascular catheters, this approach only allows mapping and ablation on the endocardial surface of the heart. Some arrhythmias, such as VT in patients with nonischemic cardiomyopathy, can arise from an epicardial focus or reentrant circuit, and are best treated by epicardial ablation, applying energy to the outer surface of the heart. Previously, this required sternotomy or thoracotomy by a cardiac surgeon. However, the technique of percutaneous subxiphoid puncture, described by Sosa et al. (1996), allows cardiac electrophysiologists to access the pericardial space for epicardial catheter ablation. However, in patients with prior history of open heart surgery, presence of adhesions in the pericardial space may preclude unrestricted catheter manipulation in the pericardial space. In such cases, a hybrid procedure in collaboration with cardiac surgeons may be required when a surgeon creates a small subxyphoid pericardial window or performs mini thoracotomy. This allows for epicardial mapping and ablation with the catheter inserted through the surgical access site that is closed by the end of the
case. Newer minimally invasive surgical epicardial ablation techniques have also been developed, and may turn out to be particularly useful for treatment of atrial fibrillation.
case. Newer minimally invasive surgical epicardial ablation techniques have also been developed, and may turn out to be particularly useful for treatment of atrial fibrillation.
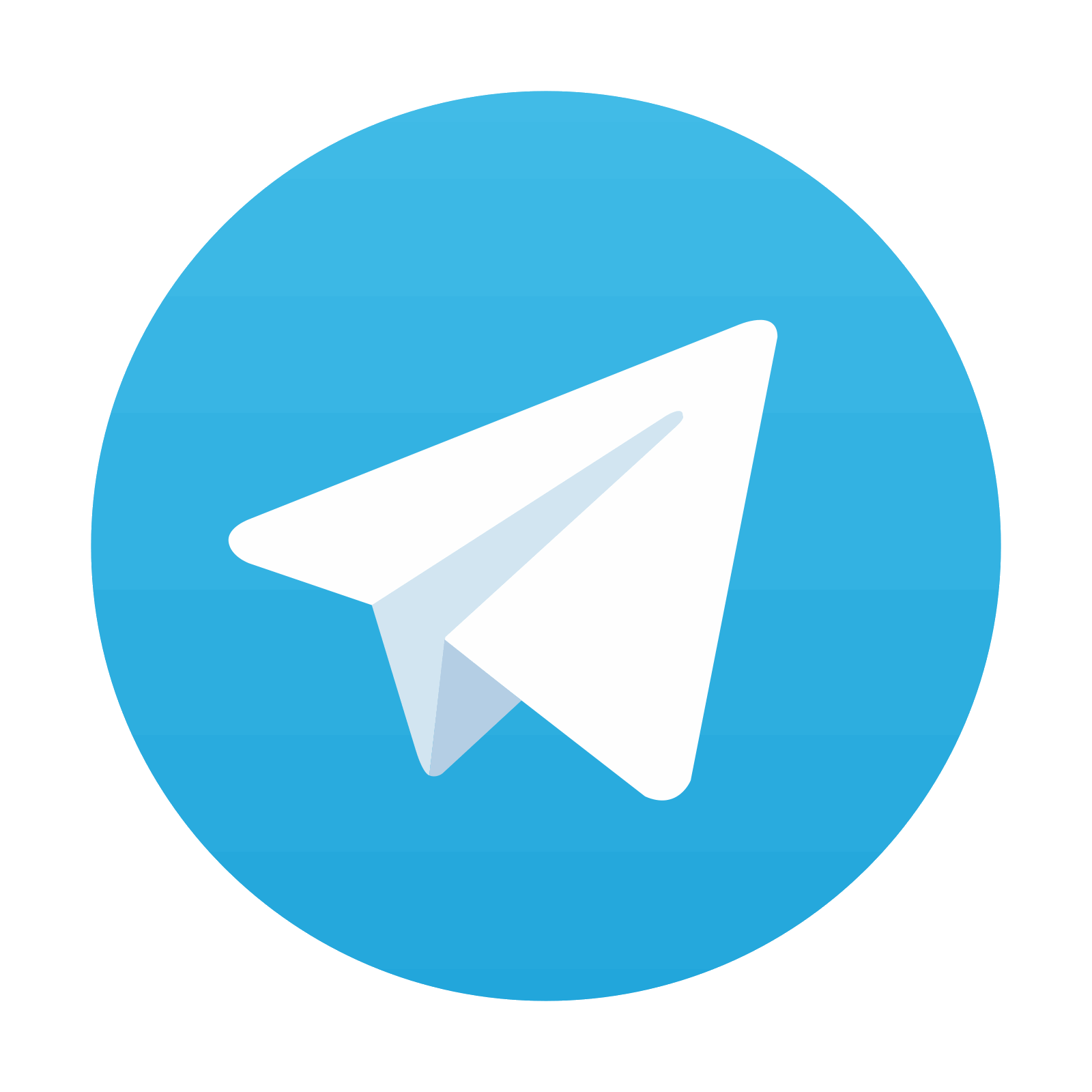
Stay updated, free articles. Join our Telegram channel
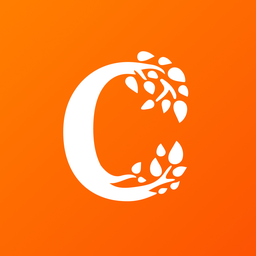
Full access? Get Clinical Tree
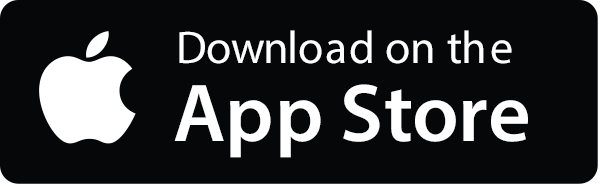
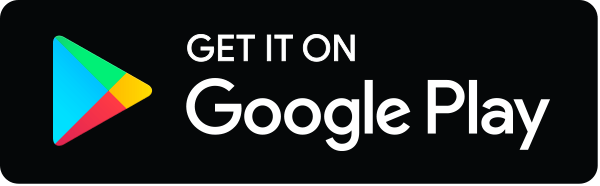