Background
Noninvasive diagnostic tools for right ventricular (RV) dysfunction measurements are increasingly being used, although their association with the pathologic mechanisms of dysfunction is poorly understood. Although investigations have focused mainly on RV systolic function, RV diastolic function remains mostly neglected. The aim of this study was to test which echocardiographic parameters best reflect RV diastolic function in mice.
Methods
Pulmonary artery banding (PAB) was used to induce RV pressure overload in mice. Transthoracic echocardiography and invasive hemodynamic measurements were performed after 3 weeks in PAB and sham-operated mice. Subsequently, the hearts were investigated by histology and analyzed for gene expression.
Results
PAB-induced pressure overload (RV systolic pressure PAB 52.6 ± 11.8 mm Hg vs sham 27.0 ± 2.7 mm Hg) resulted in RV hypertrophy and remodeling, as reflected by increased Fulton index (PAB 0.37 ± 0.05 vs sham 0.25 ± 0.02, P = .001). Masson’s trichrome staining revealed increased interstitial fibrosis (PAB 12.25 ± 3.12% vs sham 3.97 ± 1.58%, P = .002). This was associated with significant systolic RV dysfunction as demonstrated by reduced contractility index and diastolic dysfunction as demonstrated by end-diastolic pressure (PAB 2.66 ± 0.83 mm Hg vs sham 1.49 ± 0.50 mm Hg, P < .001) and τ (PAB 40.0 ± 16.1 msec vs sham 13.0 ± 3.5 msec, P < .001). Messenger ribonucleic acid expression of β-myosin heavy chain, atrial and brain natriuretic peptides, collagen family members was elevated, and the sarco/endoplasmic reticulum Ca 2+ -ATPase was decreased. Echocardiography revealed significant increases in RV free wall thickness and isovolumic relaxation time and a decrease in left ventricular eccentricity index, E′, and tricuspid annular plane systolic excursion. Isovolumic relaxation time and E′ were significantly correlated with end-diastolic pressure ( rs = 0.511 and −0.451) and τ ( rs = 0.739 and −0.445, respectively). Moreover, E′ was negatively correlated with the degree of RV fibrosis ( rs = −0.717).
Conclusions
Within 3 weeks, PAB causes pressure overload–induced RV hypertrophy and remodeling with compensated systolic and diastolic dysfunction in mice. RV free wall thickness, tricuspid annular plane systolic excursion, E′, E/E′ ratio, and isovolumic relaxation time appear to be the most reliable echocardiographic parameters for the assessment of RV dysfunction.
Highlights
- •
Assessment of RV function by echocardiography was validated.
- •
Echocardiography, hemodynamics, and morphologic analysis were directly compared.
- •
PAB in mice caused RV hypertrophy and fibrosis.
- •
PAB resulted in compensated systolic and diastolic dysfunction.
- •
RV function can be determined by changes in TAPSE, E′, E/E′ ratio, and IVRT.
Right ventricular (RV) functional status predicts survival of patients with pulmonary arterial hypertension (PAH) and congenital heart diseases. Echocardiography as a noninvasive tool is increasingly being used for the functional assessment of the right ventricle. However, the association of echocardiography-derived parameters with morphologic and histologic changes of the right ventricle is not well characterized. Previous investigations have focused on systolic function, but little is known about diastolic function.
In the left ventricle, diastolic dysfunction is known to be an important factor contributing to mortality. In patients with PAH, the presence of a delayed diastolic RV filling profile, suggestive of diastolic dysfunction, has been demonstrated by magnetic resonance imaging. Elevated RV filling pressure, which may be caused by RV diastolic dysfunction, predicts the risk for cardiac events. Accordingly, dilatation of the right atrium has been associated with adverse clinical outcomes. However, the pathologic mechanisms underlying development and progression of RV diastolic dysfunction are poorly characterized, and the diagnostic tools are not well established.
Echocardiography is the technique of choice for the serial assessment of cardiac function. However, RV function is difficult to evaluate using echocardiography because of RV shape, position, and variation with respiration. Investigations of left ventricular (LV) diastolic function are facilitated by the ellipsoid shape of the left ventricle and multiple clinical studies have demonstrated robust associations of echocardiographic parameters with LV structural changes. For RV diastolic function, such tools are still missing.
In animal models, validation of echocardiography-derived parameters is possible via direct comparison with invasive measurements and morphological analysis. The feasibility of assessing RV function using echocardiography has been demonstrated in mice and rats. In mice, Doppler imaging has been used to assess RV function. In rat models of PAH, echocardiography has been applied to evaluate RV systolic function, while diastolic function has not been addressed. Thus, the validation of the described Doppler parameters for the assessment of RV diastolic function in mice has not been performed. We hypothesized that by comparing echocardiography-derived parameters with invasive hemodynamic measurements, gene expression, and morphologic analysis, the echocardiographic parameters that best reflect diastolic RV function could be identified. To this end, RV remodeling was induced in an experimental mouse model of chronic RV pressure overload via pulmonary arterial banding (PAB).
Methods
Animal experiments were approved by the Austrian Ministry of Education, Science and Culture according to the regulations for animal experimentation (BMWF-66.010/0057-II/3 b/2012 and BMWFW-66.010/0074-WF/II/3 b/2014). The study conformed to the directive of the European Commission on the protection of animals used for scientific purposes (EU Directive 2010/63/EU). C57BL/6J male mice 11 to 12 weeks of age were obtained from Charles River, Germany. Mice were housed with a 12 h/12 h light/dark cycle, at a constant room temperature of 22°C, with access to standard laboratory chow and water ad libitum.
Animal Model
The PAB surgery was performed as previously described and in detail in the Appendix . Three weeks after surgery, mice underwent echocardiographic investigation followed by invasive hemodynamic measurements. After completion of the study, heart tissue samples were collected for histologic and molecular analysis. From a subgroup of mice, hearts were separated into the right and left ventricles and used for RV/(LV + septum) ratio measurements. A detailed description of the experimental plan is provided in Supplemental Figure 1S .
Echocardiography
A Vevo 770 High Resolution Imaging System with a 30-MHz RMV-707B scan head (VisualSonics, Toronto, ON, Canada) was used for transthoracic echocardiographic measurements. Investigations were performed under mild anesthesia with isoflurane (0.8%–1.2%) as previously described and wherever possible in accordance with the guidelines for echocardiographic assessment of the right hearts. A detailed description is provided in the Appendix .
Hemodynamic Measurements
RV systolic pressure (RVSP), blood pressure, and LV systolic pressure were measured under isoflurane anesthesia (2%–4%) using a closed-chest technique, as described previously. Briefly, a 1.4-F pressure catheter (SPR-671; Millar Instruments, Houston, TX) was inserted into the right jugular vein and directed to the right ventricle to measure RVSP. Systemic measurements were obtained via catheterization of the carotid artery. The catheter was then directed into the left ventricle to obtain LV systolic pressure. Measurements were performed and recorded for ≥5 min. Analysis was performed only for the pressure recordings with stable hemodynamics. Care was taken to ensure that the measurements for hemodynamics and echocardiography were made at similar heart rates. Online tracings and analysis were made using LabChart 7 software (ADInstruments, Spechbach, Germany). We calculated +dP/dt, −dP/dt, end-diastolic pressure (EDP), pressure-time index, and τ from pressure tracings using software analysis module. We calculated (+dP/dt)/Pmax and (−dP/dt)/P as described previously. Hemodynamic measurements were performed by experienced personnel blinded to the experimental group.
Tissue Collection and Analysis
After invasive hemodynamic measurements, animals were immediately sacrificed, and tissue samples were collected for gene expression and histologic analysis. Briefly, the chest was opened and the heart and lungs were flushed with ice-cold phosphate-buffered saline. The lungs and heart were excised, the atria were removed, and the right ventricle was separated from the left ventricle and septum. Tissue pieces were weighed and then snap-frozen in liquid nitrogen and stored at −80°C until analysis. For immunohistochemical analysis, the hearts were removed, fixed in formalin, and embedded in paraffin. For determination of congestion, internal organs (lungs, kidneys, and livers) were removed and weighed immediately. Values are presented after normalization to tibia length. No difference in tibia length was observed. For wet-to-dry ratio measurements, pieces of organs were weighed and then kept at 40°C for 2 to 3 weeks and reweighed until stable values were obtained.
Morphologic Analysis
For assessment of fibrosis, formalin-fixed, paraffin-embedded heart tissue was cut in 2-μm-thick sections and stained for collagen content using Masson’s trichrome stain according to standard protocols (red, tissue; black, nuclei; blue, collagen). Slides were scanned (Scanscope T3; Aperio, Oxford, United Kingdom) and the level of fibrosis was quantified using semiautomated image analysis (Visiomorph DP software; Visiopharm, Horsholm, Denmark). The percentage fibrosis in the right ventricle was calculated by dividing the total area of collagen (fibrosis, blue staining) by the total analyzed tissue area multiplied by 100.
For quantification of cardiomyocyte cross-sectional area, formalin-fixed, paraffin-embedded heart tissue was cut in 2-μm-thick sections and stained for laminin (Abcam, Cambridge, United Kingdom). Staining was detected using AlexaFluor Dye 555 conjugated secondary antibody (Life Technologies, Amstetten, Austria). Nuclei were counterstained using 4′,6-diamidino-2-phenylindole dihydrochloride (Sigma-Aldrich, St Louis, MO). Slides were scanned (Olympus BX61US; Olympus, Melville, NY) and obtained images were analyzed using ImageJ software (National Institutes of Health, Bethesda, MD). In the inner layer of RV myocardium, 20 to 25 cells were identified as a nucleus containing areas of laminin staining with clearly identifiable borders.
Gene Expression Analysis
Total RNA was isolated from RV tissue samples using the peqGOLD Total RNA kit (PeqLab, Erlangen, Germany) and reverse-transcribed using the iScript kit (BioRad, Hercules, CA) according to the manufacturer’s instructions. Quantitative real-time polymerase chain reaction was performed using the QuantiFast SYBR PCR kit (Qiagen, Hilden, Germany) on a LightCycler 480 (Roche, Mannheim, Germany). Expression data are normalized to the reference genes β 2 -microglobulin and porphobilinogen deaminase using the following formula ΔCt = Ct reference gene − Ct gene of interest. Data are presented as ΔΔCt (ΔCt PAB sample − mean ΔCt of sham group). Higher ΔΔCt values correspond to higher relative gene expression. All samples were assayed in duplicate, and the average value was used for quantification. Primer sequences are given in Supplemental Table 2S .
Statistical Analysis
Echocardiographic measurements were performed by B.E., and data were saved for offline analysis. Analysis was done by two investigators (B.E. and S.C.). Primary variables were pressure gradient across the clip, RV free wall (RVFW) thickness, and LV eccentricity index. For calculation of variability, five mice were randomly selected from the PAB group. For the determination of intraobserver variability, B.E. analyzed data with reanalysis after 2 to 3 weeks. To determine interobserver variability, S.C. analyzed data from the same mice, and his results were compared with those of B.E. To ensure blinding, results of analysis were not saved on original recording files. Variability was defined as the absolute difference between two sets of measurements divided by the mean of the measurements and expressed in percentage form. Intraobserver variability of B.E. for pressure gradient across the clip was 1.5%, for RVFW thickness was 9.7%, and for LV eccentricity index was 3.7%. Interobserver variability for these parameters was 8.3%, 14.0%, and 4.1%, respectively. Results presented in this report were analyzed by B.E. Data are expressed as mean ± SD. Statistical analysis was performed using SPSS version 21 (SPSS, Inc, Chicago, IL). Mann-Whitney tests were used for intergroup comparisons. Correlation analysis was performed using Spearman’s nonparametric test. Correlation analysis included data from both experimental groups. P values < .05 were considered to indicate statistical significance.
Results
Structural and Functional Changes in the Right Ventricle Due to PAB
Three weeks after operation, the PAB and sham-operated mice were investigated by echocardiography and subsequently by right-heart catheterization. Echocardiographic characterization revealed increased RVFW thickness, enlarged right ventricle, and reduced LV eccentricity index ( Table 1 and Figure 1 ). Tricuspid annular plane systolic excursion (TAPSE) and S′ were significantly reduced in the PAB group ( Table 1 ). Isovolumic contraction time was shortened and isovolumic relaxation time (IVRT) prolonged in the PAB group, resulting in similar Tei index values between the groups. The amplitude of the E wave was not significantly changed, and the amplitude of the A wave was elevated in PAB mice. The resulting E/A ratios were not different between the groups. Significant reductions of E′ in PAB mice were found by Doppler tissue imaging (DTI). There were no differences in the amplitude of A′ between the groups ( Table 1 ). Cardiac index was reduced in PAB mice ( Table 1 ). Right-heart catheterization revealed elevated RVSP, +dP/dt, and pressure-time index in PAB mice, confirming the increased RV afterload ( Table 2 ). Additionally, (+dP/dt)/Pmax, a parameter reflecting systolic function, was significantly decreased. RV EDP was elevated and τ prolonged in PAB mice, indicating diastolic dysfunction, although (−dP/dt)/P was unchanged. By gross morphologic measurements, a significant increase in the RV/(LV + septum) ratio was observed ( Figure 2 A). No signs of congestion were observed, as demonstrated by similar liver weights and lung and liver wet-to-dry ratios ( Figures 2B–2D ).
Sham ( n = 13) | PAB ( n = 12) | P | |
---|---|---|---|
Body weight (g) | 27.1 ± 1.3 | 26.8 ± 1.7 | .661 |
Heart rate (beats/min) | 409 ± 51 | 419 ± 27 | .580 |
RVFW thickness (mm) | 0.21 ± 0.06 | 0.43 ± 0.12 | <.001 |
RVIDd (mm) | 1.67 ± 0.41 | 2.58 ± 0.38 | <.001 |
D1 (mm) | 4.18 ± 0.17 | 3.25 ± 0.4 | <.001 |
D2 (mm) | 4.53 ± 0.18 | 4.35 ± 0.47 | .201 |
Eccentricity index (D1/D2) | 0.93 ± 0.04 | 0.70 ± 0.05 | <.001 |
TAPSE (mm) | 1.02 ± 0.1 | 0.60 ± 0.19 | <.001 |
Gradient velocity (mm/sec) | 668 ± 94 | 2,877 ± 372 | <.001 |
E (mm/sec) | 227 ± 92 | 316 ± 142 | .097 |
A (mm/sec) | 412 ± 51 | 504 ± 202 | .007 |
E/A ratio | 0.50 ± 0.11 | 0.57 ± 0.26 | .471 |
ET (msec) | 61.1 ± 3.6 | 71.2 ± 6.3 | <.001 |
TCOT | 81.2 ± 4.7 | 90.6 ± 9.0 | .008 |
IVCT (msec) | 11.3 ± 1.2 | 8.3 ± 0.9 | <.001 |
IVRT (msec) | 8.8 ± 3.0 | 16.4 ± 4.3 | <.001 |
Tei index | 0.33 ± 0.05 | 0.27 ± 0.11 | .138 |
E′ (mm/sec) | 22.2 ± 5.4 | 15.8 ± 4.2 | .007 |
A′ (mm/sec) | 29.3 ± 7.6 | 26.1 ± 9.0 | .37 |
S′ (mm/sec) | 27.5 ± 4.1 | 19.8 ± 4.1 | <.001 |
E/E′ ratio | 10.5 ± 3.4 | 20.2 ± 8.8 | .003 |
CI (mL/min/g) | 0.61 ± 0.11 | 0.48 ± 0.07 | .003 |

Variable | Sham ( n = 13) | PAB ( n = 12) | P |
---|---|---|---|
Heart rate (beats/min) | 469 ± 44 | 471 ± 50 | .936 |
RVSP (mm Hg) | 27.0 ± 2.7 | 52.6 ± 11.8 | <.001 |
RV EDP (mm Hg) | 1.49 ± 0.5 | 2.66 ± 0.83 | <.001 |
Pressure-time index (mm Hg · sec) | 1.13 ± 0.3 | 2.01 ± 0.51 | <.001 |
+dP/dt (mm Hg/sec) | 1,915 ± 250 | 3,133 ± 852 | <.001 |
(+dP/dt)/Pmax (1/sec) | 70.9 ± 6.2 | 59.4 ± 9 | <.001 |
−dP/dt (mm Hg) | −1,751 ± 300 | −3,036 ± 814 | <.001 |
−dP/dt/P (1/sec) | −64.8 ± 9.2 | −57.5 ± 8.6 | .036 |
τ (msec) | 13.0 ± 3.5 | 40.0 ± 16.1 | <.001 |

Pressure Overload–Induced RV Remodeling
We next investigated how the physiologic alterations in the right ventricle were reflected by gene expression changes of several molecular pathways representing diverse aspects of cardiac remodeling. Consistent with myocardial remodeling, decreased expression of α-Mhc (myosin heavy chain) and increased β-Mhc was detected ( Figures 3A and 3B ). Furthermore, increased messenger ribonucleic acid expression of atrial and brain natriuretic peptides was detected in the RV tissue ( Figures 3C and 3D ). Analysis of expression of Ca 2+ -handling proteins demonstrated downregulation of Serca2 and phospholamban but no changes in Ryr2 expression ( Figures 3E–3G ). Compared with sham mice, the messenger ribonucleic acid levels of collagen types 1a1 and 3a1 as well as connective tissue growth factor were significantly elevated in the right ventricles of PAB mice ( Figures 3H–3J ). Masson’s trichrome staining revealed significantly increased levels of RV fibrosis in PAB mice ( Figures 4A–4C ), which was restricted to the right ventricle only ( Supplemental Figure 2S ). Laminin staining revealed increased cross-sectional area of RV cardiomyocytes in PAB mice ( Figures 4D–4F ).


Correlation between Parameters of RV Function and Morphologic Changes
TAPSE and S′ have been suggested for quantification of ventricular contractile function and were strongly correlated with each other ( Supplemental Figure 3S ). Both TAPSE and S′ were negatively correlated with +dP/dt ( Supplemental Table 2S ), reflecting the afterload dependency of these parameters. TAPSE was also moderately positively correlated ( rs = 0.499, P = .011) with the contractility index, suggesting that it indicates systolic function and thus the adaptation to the increased afterload. However, TAPSE was even more strongly negatively correlated with EDP ( rs = −0.574, P = .003) and τ ( rs = −0.674, P < .001), suggesting that decreased TAPSE indicates diastolic dysfunction. Moreover, TAPSE was significantly negatively correlated with the expression level of Col1a1 and Col3a1 ( rs = −0.636 and rs = −0.729; Supplemental Table 2S ). In line with this observation, TAPSE was strongly negatively correlated with the percentage of fibrosis ( rs = −0.755, P = .007; Supplemental Table 2S and Supplemental Figure 3S ).
Among the tested echocardiographic parameters describing diastolic function, E′, E/E′ ratio, and IVRT were significantly correlated with invasively measured parameters of diastolic function EDP and τ ( Figures 5A–5C and 5E–5G ). E′ and the E/E′ ratio and IVRT were significantly correlated with the level of Col1a1 ( Supplemental Table 2S and Figures 5I and 5J ). Like TAPSE, E′ was also strongly negatively correlated with the percentage of fibrosis ( rs = −0.717, P = .03; Supplemental Table 2S ).

RVFW thickness estimated by echocardiography was very strongly correlated with RV mass normalized to LV + septum ( rs = 0.786, P = .021) or body weight ( rs = 0.952, P < .001). RVFW thickness was strongly positively correlated with RVSP ( rs = 0.630, P = .001) and moderately negatively with (+dP/dt)/Pmax ( rs = −0.526, P = .007), suggesting that increased RVFW thickness could indicate both RV afterload and systolic dysfunction ( Supplemental Table 2S ). In line with this observation, RVFW thickness was strongly correlated with TAPSE ( Supplemental Figure 3S ). RVFW thickness was also strongly correlated with EDP ( rs = 0.663, P < .001) and τ ( rs = 0.830, P < .01), suggesting that it indicates diastolic dysfunction. The correlation with Col1a1 was only moderate ( Supplemental Table 2S , Figure 5 ).
Technical Aspects of Tricuspid Flow Measurements and DTI
We observed high variability in the tricuspid inflow profile depending on respiratory cycle ( Supplemental Figure 4SA ). This resulted in significant changes in the E/A ratio depending on the respiratory phase ( Supplemental Figure 4SB ). In contrast, the DTI-derived parameters E′ and A′ showed less variation due to respiration ( Supplemental Figures 4SC and 4SD ). Both tricuspid flow and DTI demonstrated partial or complete fusion of early (E or E′) and late (A or A′) waves depending on heart rate ( Supplemental Figure 5S ). Amplitudes of A and A′ were measured at heart rates < 420 beats/min, at which they were clearly identifiable. Thus, these technical aspects interfered with measurements of deceleration time of the E wave and the E/A ratio, making them unreliable for the characterization of RV diastolic function in our model.
Results
Structural and Functional Changes in the Right Ventricle Due to PAB
Three weeks after operation, the PAB and sham-operated mice were investigated by echocardiography and subsequently by right-heart catheterization. Echocardiographic characterization revealed increased RVFW thickness, enlarged right ventricle, and reduced LV eccentricity index ( Table 1 and Figure 1 ). Tricuspid annular plane systolic excursion (TAPSE) and S′ were significantly reduced in the PAB group ( Table 1 ). Isovolumic contraction time was shortened and isovolumic relaxation time (IVRT) prolonged in the PAB group, resulting in similar Tei index values between the groups. The amplitude of the E wave was not significantly changed, and the amplitude of the A wave was elevated in PAB mice. The resulting E/A ratios were not different between the groups. Significant reductions of E′ in PAB mice were found by Doppler tissue imaging (DTI). There were no differences in the amplitude of A′ between the groups ( Table 1 ). Cardiac index was reduced in PAB mice ( Table 1 ). Right-heart catheterization revealed elevated RVSP, +dP/dt, and pressure-time index in PAB mice, confirming the increased RV afterload ( Table 2 ). Additionally, (+dP/dt)/Pmax, a parameter reflecting systolic function, was significantly decreased. RV EDP was elevated and τ prolonged in PAB mice, indicating diastolic dysfunction, although (−dP/dt)/P was unchanged. By gross morphologic measurements, a significant increase in the RV/(LV + septum) ratio was observed ( Figure 2 A). No signs of congestion were observed, as demonstrated by similar liver weights and lung and liver wet-to-dry ratios ( Figures 2B–2D ).
Sham ( n = 13) | PAB ( n = 12) | P | |
---|---|---|---|
Body weight (g) | 27.1 ± 1.3 | 26.8 ± 1.7 | .661 |
Heart rate (beats/min) | 409 ± 51 | 419 ± 27 | .580 |
RVFW thickness (mm) | 0.21 ± 0.06 | 0.43 ± 0.12 | <.001 |
RVIDd (mm) | 1.67 ± 0.41 | 2.58 ± 0.38 | <.001 |
D1 (mm) | 4.18 ± 0.17 | 3.25 ± 0.4 | <.001 |
D2 (mm) | 4.53 ± 0.18 | 4.35 ± 0.47 | .201 |
Eccentricity index (D1/D2) | 0.93 ± 0.04 | 0.70 ± 0.05 | <.001 |
TAPSE (mm) | 1.02 ± 0.1 | 0.60 ± 0.19 | <.001 |
Gradient velocity (mm/sec) | 668 ± 94 | 2,877 ± 372 | <.001 |
E (mm/sec) | 227 ± 92 | 316 ± 142 | .097 |
A (mm/sec) | 412 ± 51 | 504 ± 202 | .007 |
E/A ratio | 0.50 ± 0.11 | 0.57 ± 0.26 | .471 |
ET (msec) | 61.1 ± 3.6 | 71.2 ± 6.3 | <.001 |
TCOT | 81.2 ± 4.7 | 90.6 ± 9.0 | .008 |
IVCT (msec) | 11.3 ± 1.2 | 8.3 ± 0.9 | <.001 |
IVRT (msec) | 8.8 ± 3.0 | 16.4 ± 4.3 | <.001 |
Tei index | 0.33 ± 0.05 | 0.27 ± 0.11 | .138 |
E′ (mm/sec) | 22.2 ± 5.4 | 15.8 ± 4.2 | .007 |
A′ (mm/sec) | 29.3 ± 7.6 | 26.1 ± 9.0 | .37 |
S′ (mm/sec) | 27.5 ± 4.1 | 19.8 ± 4.1 | <.001 |
E/E′ ratio | 10.5 ± 3.4 | 20.2 ± 8.8 | .003 |
CI (mL/min/g) | 0.61 ± 0.11 | 0.48 ± 0.07 | .003 |
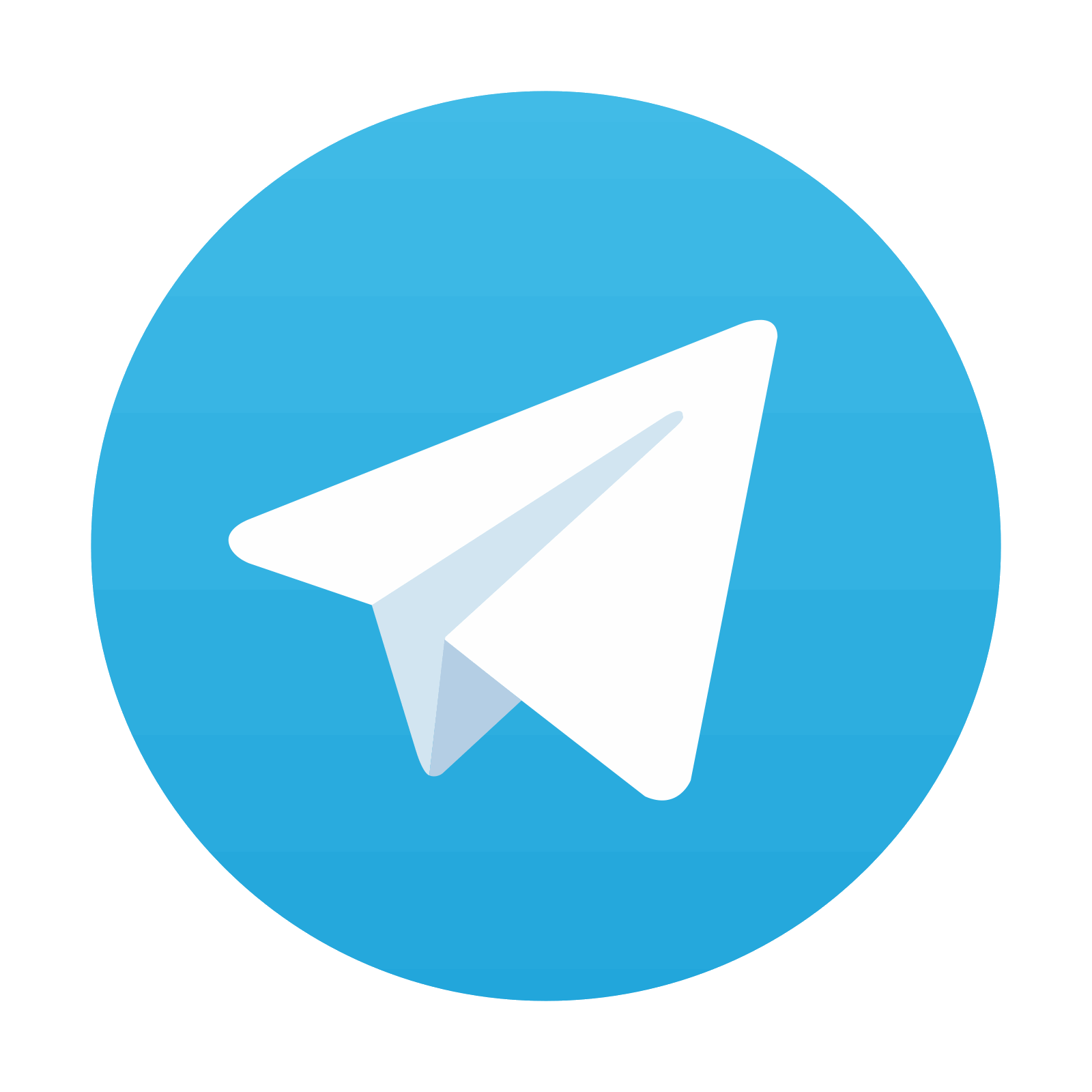
Stay updated, free articles. Join our Telegram channel
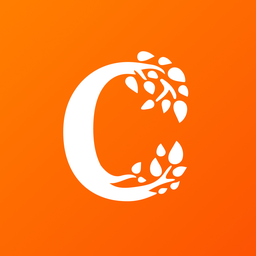
Full access? Get Clinical Tree
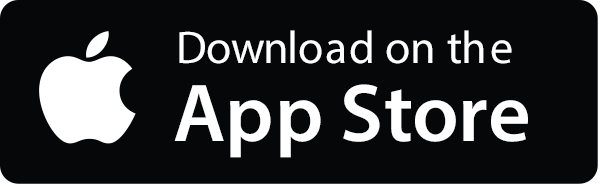
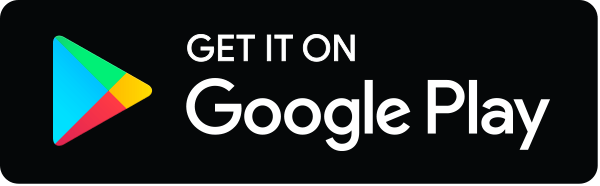
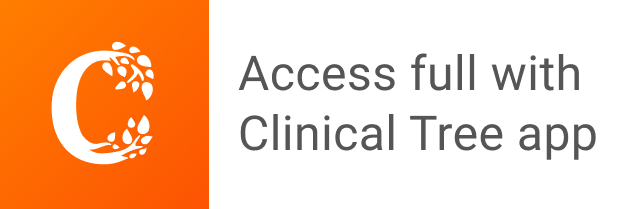