Plaque Characterization— Computed Tomography
Hans-Christoph Becker
DEVELOPMENT OF CORONARY ATHEROSCLEROSIS
Coronary atherosclerosis begins as early as the first decade of life with endothelial dysfunction, proliferation of smooth muscle cells, and deposition of fatty streaks in the coronary artery wall (1). At the later stage of this still clinically silent disease, these lesions may further accumulate cholesterol within the intima and media coronary artery wall layer, with a fibrous cap separating the lipid pool from the coronary artery lumen (2). Inflammatory processes with invasion of macrophages and activation of matrix metalloproteases may cause consecutive weakening of the fibrous cap (3).
Such vulnerable plaques can rupture when exposed to shear stress, and the thrombogenic lipid material may enter the bloodstream. In the most unfortunate event, thrombus progression turns the vulnerable plaques into culprit lesions that occlude the coronary vessels, leading to myocardial ischemia, ventricular fibrillation, and death (4). Alternatively, studies show that plaque erosions with consecutive thrombus formation constitute approximately 40% of cases of sudden coronary death (5). Plaque erosions are more commonly seen in young women and men of 50 years of age, and are associated with smoking, especially in premenopausal women.
In the initial stadium of atherosclerosis, the coronary vessel widens at the location of the atherosclerotic plaques. The phenomenon is called “positive remodeling” and explains why such plaques may not be seen by cardiac catheter (6) (Fig. 28.1). Nonfatal plaque rupture or erosion at end stage may heal, organize, and subsequently calcify. Consecutively, fibro-calcified lesions may reduce vessel lumen diameter (negative remodeling), with consecutive reduction of blood flow and myocardial ischemia. In clinical terms development of fibro-calcified plaques may lead to stable and chronic angina.
ESTIMATION OF CARDIAC EVENT RISK
In many patients, unheralded myocardial infarction (MI) associated with a mortality of approximately 20% is the first sign of coronary artery disease (CAD). The risk of an event strongly depends on risk factors such as hypertension, hypercholesterolemia, smoking habits, family history, age, and gender. Based on these risk factors, algorithms such as the Framingham (7), PROCAM (8), and SCORE (9) provide an estimation of the midterm (10-year) risk for an individual subject to experience a cardiac event. According to most of the international guidelines, patients with a midterm risk of less than 10% are considered to be at low risk and usually require advice for a healthy lifestyle but no specific therapy. Patients with a midterm risk of more than 20% are considered to be at high risk and, therefore, may be termed patients with a CAD equivalent. Similar to patients with established CAD, these asymptomatic patients may require intensive intervention for risk reduction, such as lifestyle changes and lifetime medical treatment.
Approximately 40% of the population is considered to have a moderate (10% to 20%) midterm risk of CAD. All of the currently available risk stratification schemes suffer from the lack of accuracy to correctly determine the risk, and uncertainty exists as to how to treat patients identified as intermediate risk. Providing information to reassure and/or treat intermediate-risk patients is a high priority. Currently, besides testing for myocardial ischemia (e.g., by treadmill testing), assessment of the atherosclerotic plaque burden provides valid information for further risk stratification in patient cohorts (10).
Tests for myocardial ischemia, such as electrocardiographic (ECG) stress testing, are better suited to diagnose patients with ischemic CAD than to assess clinically silent atherosclerosis in the coronary arteries. Determination of the intima-media thickness (IMT) and ankle bracelet index (ABI) by ultrasound and Doppler focus on the assessment of the atherosclerotic plaque burden in the carotid and peripheral arteries, respectively. However, only computed tomography (CT) and magnetic resonance imaging (MRI) have the ability to noninvasively assess the extent of the atherosclerotic plaque burden in the coronary arteries. MRI is superior to CT in terms of soft-tissue differentiation (11). However, CT is currently superior to MRI in terms of spatial and temporal resolution to image the small and constantly moving structures such as the coronary arteries. Therefore, CT is the only reliable and practicable tool to investigate the entire coronary artery tree and to quantify the atherosclerotic plaque burden noninvasively (12).
TABLE 28.1 Acquisition Parameters for Computed Tomography | |||||||||||||||||||||||||||||||||||||||||||||
---|---|---|---|---|---|---|---|---|---|---|---|---|---|---|---|---|---|---|---|---|---|---|---|---|---|---|---|---|---|---|---|---|---|---|---|---|---|---|---|---|---|---|---|---|---|
|
COMPUTED TOMOGRAPHY TECHNIQUE
Initially, coronary CT was performed with electron beam CT (EBCT) since lack of any moving items allowed for exposure times as short as 100 milliseconds. Scan acquisition in EBCT is triggered by the ECG signal to the mid-diastole phase of the cardiac cycle to further reduce cardiac motion artifacts (Table 28.1). The initial intent of EBCT was to measure myocardial perfusion. However, EBCT can also detect coronary calcifications as a surrogate marker for coronary atherosclerosis (13). Further attempts have also been made to
use this scanner for CT angiography (CTA) and plaque imaging of the coronary arteries. However, low spatial resolution (3 mm) and high image noise of EBCT limits the investigation to the most proximal part of the coronary arteries (14). Conventional mechanical CT scanners with an x-ray tube and detector ring rotating around the patient have also increased in speed within recent years. The scan mode dedicated to coronary multidetector-row (MD) CT is called retrospective ECG gating (15). With this technique, the spiral CT scan is acquired with a small pitch (table feed per gantry rotation), and image reconstruction is performed in the slow motion diastolic phase of the cardiac cycle. Coronary calcium quantities measured with the first clinical available MDCT scanner with four detector rows and temporal resolution of 250 milliseconds are well comparable to EBCT (16,17).
use this scanner for CT angiography (CTA) and plaque imaging of the coronary arteries. However, low spatial resolution (3 mm) and high image noise of EBCT limits the investigation to the most proximal part of the coronary arteries (14). Conventional mechanical CT scanners with an x-ray tube and detector ring rotating around the patient have also increased in speed within recent years. The scan mode dedicated to coronary multidetector-row (MD) CT is called retrospective ECG gating (15). With this technique, the spiral CT scan is acquired with a small pitch (table feed per gantry rotation), and image reconstruction is performed in the slow motion diastolic phase of the cardiac cycle. Coronary calcium quantities measured with the first clinical available MDCT scanner with four detector rows and temporal resolution of 250 milliseconds are well comparable to EBCT (16,17).
CORONARY CALCIUM SCREENING
A four detector-row CT with 500-millisecond gantry rotation time is the minimal requirement for a coronary calcium measurement. Coronary calcium screening can be performed by any MDCT without contrast media and with 3-mm slices. Overlapping slice reconstruction improves reproducibility of the coronary calcium quantification (17). Retrospective ECG gating is superior in acquiring the entire volume without any gaps and reconstructing the images with overlapping increment. An overlapping slice reconstruction may help to improve the reproducibility (18). Depending on the MDCT scanner, the scan time may range from 5 to 20 seconds, and the entire investigation is completed within 5 minutes. In total, 40 to 80 slices are generated with one investigation.
As a fundamental requirement for screening, the radiation exposure for coronary calcium scanning needs to be reduced to a minimum (approximately 1 mSv). In particular, when assessing coronary atherosclerosis in asymptomatic patients, redundant radiation should be avoided. On the base of the ECG signal, the x-ray tube current is switched to its nominal value during the diastolic phase and is reduced significantly during the systolic phase of the heartbeat. This technique is called prospective ECG tube current modulation or ECG pulsing, and is most effective in patients with low heart rates. If the heart rate is lower than 60 bpm, the radiation exposure will be reduced by approximately 50% (19).
Alternatively, prospective ECG triggering is a radiation dose efficient scan mode for coronary calcium screening, if exposure is dedicated to the time point of the least cardiac motion only. Newest generation dual source CT allows for ECG triggered high pitch scan acquisition. This scan mode has proved to be the most efficient in terms of radiation exposure. However, in higher heart rate image quality may be degraded by heart motion and small calcifications may then be missed with this technique.
One of the other prerequisites is constant kVp of the x-ray tube. The photon energy of the x-ray beam has pivotal impact on the density of coronary calcium in the CT image. Most modern CT scanners allow for 120 kVp tube voltage that is closest to the scan protocol with the EBCT.
Even the smallest calcifications will become visible by the reconstruction with a no-edge enhancing soft-tissue kernel. After the reconstruction, the image data are analyzed and postprocessed by a dedicated workstation. Upon identification of the specific lesions, the workstation automatically displays the quantities of coronary calcium as the Agatston score, volume equivalent, and absolute mass (Fig. 28.2). According to the method proposed by Agatston, any dense structure in the CT image greater than 130 HU in density is identified as calcification. The area of this calcification is multiplied by a factor that depends on the peak density of the lesion. A factor between 1 and 4 is used for a peak density of 130 to 199 HU, 200 to 299 HU, 300 to 399 HU, and greater than 400 HU, respectively. The sum of all lesions in all four coronary vessels (left main, left anterior descending, circumflex, and right coronary artery) corresponds to the total Agatston score (13). This algorithm requires specific EBCT image quality and nonoverlapping slice thickness of 3 mm. Therefore, the Agatston quantification method is of limited value for MDCT.
Originally, the first investigations with the EBCT allowed for acquisition of half of the entire heart because no more than 20 slices could be acquired at once. With this approach, the limited reproducibility became obvious (20), and a number of authors have suggested algorithms to improve the reproducibility. To allow for a reproducible measurement of coronary calcium for follow-up investigations, it was necessary to introduce the calcium volume equivalent (19). With isotropic interpolation, overlapping slice acquisition can be simulated by a workstation, and the reproducibility of the quantification can be improved. However, real overlapping slice acquisition is always superior to isotropic interpolation (21) and should be used with MDCT. The volume score, as well as the Agatston score, is limited since the quantity of the calcium volume depends on the image quality and the values cannot easily be transferred from EBCT to MDCT.
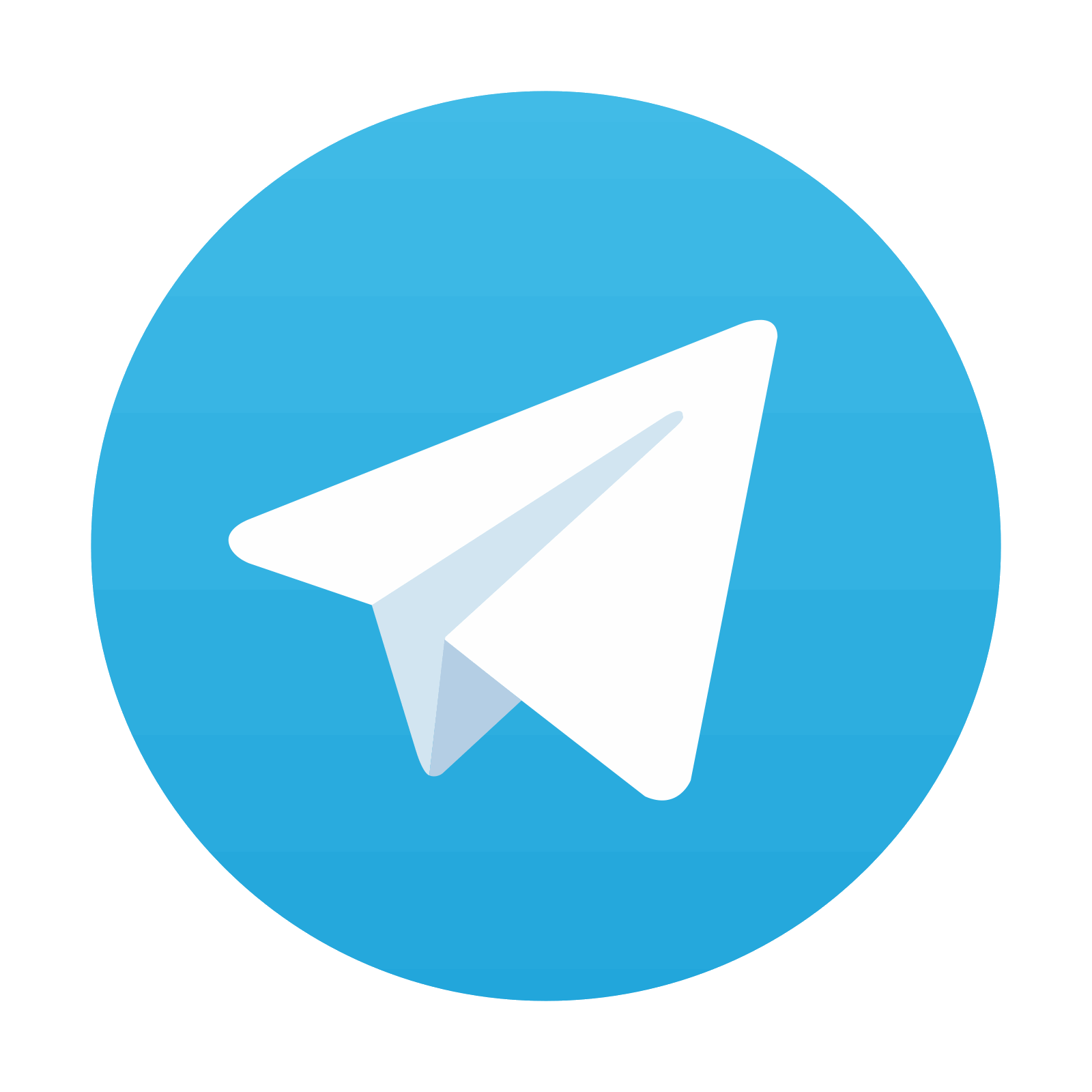
Stay updated, free articles. Join our Telegram channel
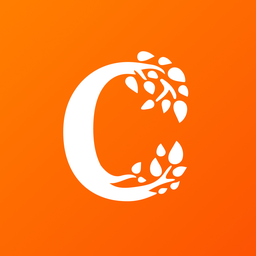
Full access? Get Clinical Tree
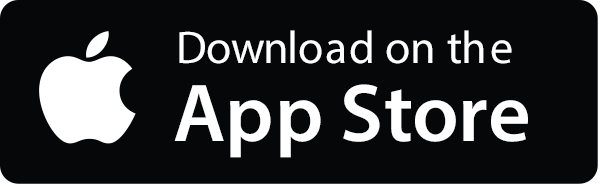
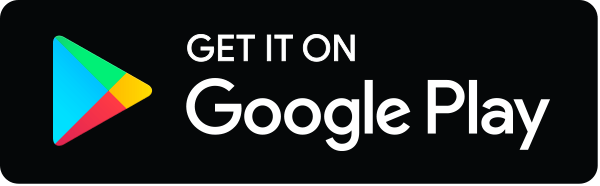