Fig. 8.1
Membrane currents that generate the normal action potential. Resting (4), upstroke (0), early repolarization (1), plateau (2), and final repolarization are the 5 phases of the action potential. A decline of potential at the end of phase 3 in pacemaker cells, such as the sinus node, is shown as a broken line. The inward currents, INa, ICa, and If, are shown in yellow boxes; the sodium-calcium exchanger (NCX) is also shown in yellow. It is electrogenic and may generate inward or outward current. IKAch, IK1, Ito, IKur, IKr, and IKs are shown in gray boxes. The action potential duration (APD) is approximately 200 ms (Reused with permission from Grant [84])
Autonomic Physiology
At rest the heart receives autonomic tone from both the sympathetic and parasympathetic nervous systems. However, vagal tone predominates to suppress the resting HR. The preganglionic neurons of both the sympathetic and parasympathetic nervous systems release acetylcholine that binds to nicotinic receptors on the cell bodies of the postganglionic neurons. Postganglionic sympathetic fibers synapsing at the heart release norepinephrine which binds to type-1 beta-adrenergic receptors. Postganglionic parasympathetic fibers of the vagus nerve release acetylcholine to stimulate type-2 muscarinic receptors (M2) on the heart. Both receptors are G-protein coupled receptors. The type-1 beta receptor is a stimulatory G-protein linked receptor and the type-2 muscarinic receptor is inhibitory. G-protein dissociates upon ligand binding and either stimulates or inhibits adenylyl cyclase. This results in either increased or decreased cAMP production respectively. Increasing cAMP leads to an increase in HR and contractility and vice-versa [7]. Additionally, acetylcholine released from the parasympathetic fibers binds to ligand-gated potassium channels to decrease the rate of depolarization and slow the HR.
Both muscarinic and beta-adrenergic receptors are found on the sinus node, AV node and atria, however, only beta receptors are present on the ventricles. Thus the parasympathetic nervous system has no influence on ventricular contractility.
Homeostasis of the Cardiovascular System
Homeostasis of the cardiovascular system is controlled primarily by the baroreceptor and chemoreceptor pathways. Changes in arterial pressure, O2 concentration and to a lesser extent CO2 concentration are detected and result in appropriate autonomic responses to sustain the blood pressure in the short term [8].
Baroreceptor Reflex
A decrease in arterial pressure is detected by baroreceptors in the carotid sinus and aortic arch. They are stretch receptors that inhibit sympathetic stimulation to the heart. Signals are sent via afferent fibers of the vagus and glossopharyngeal nerves to the solitary nucleus in the medulla. When blood pressure falls, the baroreceptors detect a decrease in wall tension. The receptors in turn decrease their rate of firing that disinhibits the sympathetic nervous system resulting in an increase in total vascular resistance and HR and contractility [8].
Similar to the baroreceptor reflex is the atrial reflex (also known as the Bainbridge reflex) in which stretch receptors of the atria detect changes in venous return to the heart. An increase in venous return causes an increase in HR through the efferent limb of the reflex to the sinus node. The opposite is also true.
Chemoreceptor Reflex
Chemoreceptors located in the carotid and aortic bodies respond primarily to changes in the partial pressure of oxygen but also monitor the partial pressure of carbon dioxide and pH. Hypoxia, hypercapnia or acidosis will increase the firing rate of chemoreceptors and results in an increase in both the rate and depth of respiration. Sympathetic tone to the heart is then increased through both direct and indirect mechanisms [8]. Chemoreceptors have a direct effect on medullary vasomotor neurons supplying the heart. Indirectly, by increasing the depth of breathing, stretch receptors in the lung result in increased sympathetic stimulation to the heart [8].
Exercise and the Denervated Heart
Exercise tolerance post-transplantation is greatly improved compared with end-stage heart failure but peak oxygen uptake (VO2 max) in recipients is reported at only 50–70% of age-adjusted expected values [2]. A lower VO2 max correlates strongly with morbidity and mortality [9]. One of the goals in the long-term management of the HT patient, therefore, is to optimize exercise capacity. This lower observed exercise capacity is not only the result of the denervated heart but also due to peripheral factors. Examples include impairments to vasodilation and a decline in skeletal muscle function. These changes occur during heart failure pre-transplantation and are reversible through exercise although not entirely [10]. At levels of exercise below the maximum, appropriate cardiac outputs are observed in the HT recipient [9]. Ejection fraction and systolic function are normal during exercise. There is diastolic dysfunction that must be compensated for with higher filling pressures. Pulmonary artery wedge pressures of twice the resting value have been demonstrated during maximal exercise in HT patients [11].
Allograft Response to Exercise
Dynamic exercise requires an increase in cardiac output (CO) to meet the increased metabolic demands of skeletal muscle and maintain aerobic respiration. The two components of CO are heart rate and stroke volume (SV). The normal heart responds to exercise predominantly by increasing its HR in response to the neural and hormonal effects on the sinus and AV nodes. A decrease in vagal tone allows the HR to rise to the intrinsic rate of depolarization of the sinus node. A further increase in HR occurs due to sympathetic stimulation. This is augmented by circulating catecholamines. HR promptly drops after the cessation of exercise. During strenuous exercise, SV will also increase in the normal heart. Skeletal muscle vasodilation causes a decrease in peripheral vascular resistance, an increase in venous return and therefore an increase in SV.
In contrast, the denervated heart does increase its HR in response to exercise but more slowly and achieves a lower maximum HR. The increase in HR is in response to circulating catecholamines rather than from the effects of the autonomic nervous system [12]. HR is slower to normalize and actually peaks after exercise stops (Fig. 8.2). The transplanted heart is said to be “preload dependent” since stroke volume relies on venous return [13]. During mild exercise, left ventricular end-diastolic volume and pressure increase. This increase in venous return will further stretch the myocardial fibers leading to greater contractility (Frank-Starling’s mechanism). Over time the donor heart becomes increasingly sensitive to catecholamines [12].


Fig. 8.2
A graphic of comparison between the chronotropic response of the innervated heart versus the denervated heart (Reused with permission from Dall et al. [32])
In the normal individual isometric exercise causes muscles to produce metabolites such as lactate that stimulate the autonomic nervous system to increase the HR and constrict peripheral arterioles. The arterial blood pressure elevates and CO increases slightly [14]. The transplant patient shows a similar response to isometric exercise with a slight increase in CO and increases in systolic and diastolic arterial pressures [15]. Mechanisms controlling changes to peripheral vascular resistance remain intact. The significant difference is the lack of HR acceleration seen in normal individuals performing isometric exercise [16].
The transplanted heart is unable to reach the CO of the normal heart at rest and during exercise. Transplant patients cannot sustain exercise for as long as control individuals. Oxygen extraction is heightened, reflected in an increased arterio-venous (AV) oxygen gradient. Transplant recipients also undergo more anaerobic respiration when exercising as demonstrated by an increase in lactate concentration [17].
Peripheral Factors Affecting Exercise
Heart failure patients, especially those who ultimately undergo heart transplantation, spend prolonged periods of time in a state of deconditioning due to a decline in exercise capacity, decompensations, hospitalizations and being in a bed-ridden state. The peripheral skeletal muscles decrease in mass and on a microscopic level show fewer mitochondria and a shift towards a predominance of fast-twitch fibers, an increase in glycolytic enzymes and a decrease in oxidative enzymes and creatine kinase. As such, these muscles are preferentially glycolytic and produce more lactate [18]. Although the oxidative capacity of skeletal muscle normalizes after transplantation, the capillary beds do not regrow entirely. These persisting vascular abnormalities contribute to decreased exercise capacity post-transplantation [19].
Pulmonary function declines in severe heart failure. Although there is a marked improvement in pulmonary function tests after transplantation, the lung diffusion of carbon monoxide (DLCO) remains below the predicted value even when there is no underlying lung disease. This is because the pulmonary capillary wedge pressure is elevated in heart failure leading to the capillary endothelium becoming irreversibly damaged. In HT patients who have a DLCO of <50% of predicted, exercise results in respiratory acidosis and hypoxemia [20].
Exercise Protocols for the Heart Transplant Recipient
The chronotropic incompetence seen in HT patients improves after the first year post-transplantation with resting HR decreasing, maximum HR increasing and peak VO2 increasing even without a prescribed regimen of exercise [21]. However, the benefits of exercise were demonstrated by Kobashigawa et al. in a randomized control trial [22] that has been affirmed by numerous studies since. Increases in VO2 max after exercise regimens ranged from 13 to 28 mL/kg/min [23–29]. Patients who are motivated and follow a supervised training program show a 50% improvement in VO2 max compared with recipients who remain sedentary [19].
Previously it was thought that the loss of chronotropy due to denervation meant that exercise regimens needed to be limited to moderate training protocols. It was also thought that central factors influenced exercise capacity more than others. Evidence now suggests that peripheral factors have a larger impact on the decreased exercise capacity post-transplantation [30]. It has also been demonstrated that chronotropy can normalize both early and late post-transplantation [31, 32].
High Intensity Interval Training
With an improved understanding of the physiology of the transplanted heart and the impact of peripheral factors on exercise tolerance in the heart transplant recipient, the effectiveness of high intensity interval training (HIIT) has been demonstrated [27–29, 32]. HIIT has long been a therapeutic tool in the long-term management of cardiovascular disease and heart failure. These patients have shown a marked improvement in exercise tolerance with an increase in VO2 max of 46% and even a reversal of ventricular structural changes after 12 weeks [33].
HIIT requires the patient to engage in aerobic exercise until a VO2 of 85–95% of max or a HR of 90–95% of predicted max is achieved (see Fig. 8.3 for a comparison between HIIT and continued moderate training). This is followed by a period of rest until HR falls to 60–70% of max. The cycle is then repeated four times [34]. The transplant patient must augment this regimen with both a cool-down and a warm-up period due to chronotropic incompetence and a reliance on circulating catecholamines to increase CO as discussed earlier. Compliance is an issue when prescribing exercise protocols to patients especially when psychological co-morbidities such as depression and anxiety exist.


Fig. 8.3
The HIIT training protocol (10-min warm-up, 16 min of HIIT training [>80% of VO2peak] + 14 min of recovery and 10-min cool-down) and the CON training protocol (10-min warm-up, 45 min of CON training [~60–70% of VO2peak] and 10-min cool-down) (Reused with permission from Dall et al. [35])
HIIT improves VO2 max and more so than moderate training programs. Some patients are able to attain a VO2 max of 80–89% of predicted [29]. Systolic systemic blood pressure falls by an average of 3 mmHg. Resting HR decreases slightly with HIIT but no change is seen in patients on protocols of moderate exercise [32]. HR recovery time improves after both moderate exercise protocols and HIIT with HIIT proving slightly more beneficial. This is important because HR recovery time strongly correlates with mortality [35, 83]. Peripheral factors also show an improvement with skeletal muscle mass increasing and an increase in mitochondrial density [29]. Coronary allograft vasculopathy (CAV) remains a significant cause of mortality in HT patients. HIIT may reduce the progression of CAV, although the evidence is limited to animal models [36] and a single study in humans [37].
Improvements in VO2 max are lost after 5 months of stopping exercise regimens. Patients also show more signs of depression and anxiety, highlighting the importance of continuing exercise on a life-long basis [35].
Reinnervation
Reinnervation of the transplanted heart is a controversial topic but there is evidence that both sympathetic and parasympathetic reinnervation can occur, although it is highly variable between patients and even heterogeneous within the same patient [38]. Bengel et al. [39] in a longitudinal study, showed that complete reinnervation could take 15 years from the time of transplantation. It is uncertain whether exercise improves autonomic control or whether it occurs independently over time [24, 40]. Reinnervation is significant because resumption of chronotropic control is associated with better exercise capacity [41]. Reinnervation also allows for pain sensation such as angina [42] and improves regulation of blood flow to the myocardium [43].
Determinants of Reinnervation
The heterogeneous pattern of reinnervation [39] and regional differences in its prevalence [44] suggest that certain factors may influence whether or not reinnervation occurs. It is likely that donor age plays a role. This is possibly due to a reduction in neurotrophins. Neurotrophins are required for peripheral nerve growth and decline with age [45, 46]. Peripheral neuron axonal re-growth occurs along arteries [82]. Extensive scarring caused by increased cross-clamp times, and aortic complications negatively impact the reinnervation process. Pre-transplantation cardiac pathology is another factor. Patients who received a transplant for dilated cardiomyopathy were more likely to undergo reinnervation than those who had ischemic heart disease (IHD) [47]. This is possibly due to the sclerotic aorta seen in IHD being less amenable to nerve regrowth. Additionally, time spent on cardiopulmonary bypass correlates with the time taken for reinnervation to occur [40].
Quantifying Reinnervation
A physiological marker of autonomic innervation is heart rate variability. Due to simultaneous and varying degrees of input from the parasympathetic and sympathetic nervous systems, the HR subtly changes from contraction to contraction. Power spectrum analysis assesses heart rate variability and is non-invasive [48]. Another technique frequently employed is cardiac scintigraphy with 123I-metaiodobenzylguanidine (123I-MIBG). 123I-MIBG is radioactive, behaves like norepinephrine and is taken up by myocardial sympathetic nerve fibers. Therefore, it is assumed that positive uptake is a sign of reinnervation [49]. Alternatively, cardiac norepinephrine release can be quantified directly in response to tyramine administration but this is an invasive method [50]. Immunohistochemical studies are also used to show histological evidence of new nerves extending through sutures lines [51] (see Fig. 8.4). Positron emission tomography (PET) is a powerful but expensive method for visualizing the norepinephrine analogue 11C-hydroxyephedrine which is taken up by cardiac neurons [39]. Again, it is assumed that uptake reflects reinnervation.


Fig. 8.4
Immunohistochemical study of an endomyocardial biopsy specimen using anti-S100 antibody (×400) shows clustered nerve fibers (arrow) (Reused with permission from Gallego-Page et al. [85])
There is evidence that sympathetic reinnervation occurs in up to 40% of patients 1 year post-transplantation [4]. Denervation eliminates presynaptic sympathetic fibers and causes myocardial stores of norepinephrine to deplete [52, 53]. The reduced exercise capacity of the transplant recipient is a result of inotropic impairment as well as chronotropic incompetence.
Sympathetic reinnervation improves the chronotropic responsiveness of the heart and restores the ventricular inotropic response to exercise. The maximal HR increases and the VO2 max also rises. Patients with sympathetic reinnervation show an improved tolerance for exercise. In particular, patients with sinus node functional improvement show the greatest increase in exercise capacity. Attaining a maximal inotropic response requires local norepinephrine as well as catecholamines released by the adrenal medulla. Reinnervation results in the reappearance of presynaptic terminals and restoration of the myocardial norepinephrine stores [4]. Consequently, the inotropic response improves profoundly [54].
Chest pain from myocardial ischemia is transmitted through unmyelinated afferent fibers of the sympathetic nervous system. HT patients who undergo sympathetic reinnervation are able to experience during ischemic episodes. Those who remain denervated suffer silent ischemia [42].
Another role of the sympathetic nervous system is to regulate coronary blood flow. Even within the same patient there are prominent differences in myocardial blood flow depending on the extent of reinnervation. Areas with sympathetic reinnervation have increased blood flow [43].
Parasympathetic Reinnervation
The functional significance of parasympathetic reinnervation is unknown. The extent to which it occurs is contentious and not well defined due to difficulties in measuring parasympathetic activity in the heart. Early studies found that histological evidence of parasympathetic reinnervation only appeared 10 years after transplantation [55]. Physiological studies similarly only demonstrated parasympathetic tone after 8 years [56, 57]. These finding may be the result of surgical technique. The biatrial method was the standard until the mid-to-late 1990s until the bicaval method predominated. Parasympathetic reinnervation has been demonstrated in patients who underwent a bicaval anastomosis within a year of transplantation. This could be because the bicaval technique results in both the parasympathetic and sympathetic fibers of the recipient being dissected whereas the in the biatral technique approximately half of the sympathetic fibers are cut and the parasympathetic fibers of the recipient are left intact. Surgical dissection of nerves may stimulate axonal regrowth [58].
Heart transplant patients have markedly delayed HR recovery times. In healthy subjects HR recovery is associated with parasympathetic tone [59]. In addition to being lower than predicted, peak HR is achieved after the cessation of exercise, a feature unique to heart transplant patients. This is due to a lack of vagal innervation required to decelerate the HR and the time taken to metabolize circulating catecholamines [40]. Parasympathetic reinnervation might occur as early as 6 months post-transplantation [40] and may result in improved HR recovery times and a lower resting HR [29].
Electrophysiology of the Transplanted Heart
The transplanted heart invariably has a different electrophysiology from the normal heart. Surgical technique, denervation, ischemia, fibrosis, CAV and reinnervation all play a role in altering the conduction system, sometimes leading to clinically significant arrhythmias [60].
There is a wide range for the incidence of atrial arrhythmias post-transplantation in the literature. Atrial fibrillation (AF) is reported at 0.3–24% after HT [61–64]. Larger studies tend to report lower numbers [61, 62, 64]. Early AF is rarer after heart transplantation than after other forms of cardiac surgery such as coronary artery bypass grafting or valve replacement. This may be because the donor heart is usually healthier than the hearts of other cardiac patients.
Whether rejection causes AF or atrial flutter is equivocal. There may be an association between sustained AF or atrial flutter and episodes of rejection [61, 63–65]. Therefore, a finding of AF or atrial flutter should be investigated to exclude rejection [65]. Repeated episodes of rejection scar the atria which can lead to atrial flutter [61]. Atrial flutter is more common than AF in HT patients and usually occurs later after transplantation [63, 65]. Donor age and use of the biatrial method are risk factors [66]. Patients who develop atrial flutter are at increased risk of developing LV dysfunction and early death [61]. Once primary etiologies have been excluded, sustained atrial flutter can be treated with radiofrequency ablation.
Denervation and reinnervation impact the electrophysiology of the transplanted heart. As mentioned earlier, denervation results in a decrease in HR variability and an increase in resting HR. The corrected QT interval correlates with sympathetic reinnervation. Heterogeneous reinnervation increases the risk of ventricular arrhythmias [67].
After transplantation it is common for patients to become bradycardic as the result of sinus node ischemia [68]. A subset of these patients develops a form of sick sinus syndrome known as bradycardia-tachycardia syndrome [68]. Caution should be exercised in these patients if treating AF as some therapeutic agents can worsen bradycardia. Complete AV block manifests later on after transplantation and is probably due to progressive ischemic injury to the conduction system from CAV [69]. Approximately 10% of HT patients with bradycardia will need a permanent pacemaker [70]. This number may decline in the future because biatrial anastomosis is a major risk factor for needing a permanent pacemaker. Interestingly, needing a permanent pacemaker does not impact survival [71].
Ventricular arrhythmias are relatively common immediately following transplantation. Later on, ventricular tachycardia (VT) could be the result of CAV and should be followed up with coronary angiography and an endomyocardial biopsy [72]. The placement of an ICD may be required [73].
Improvements in the survival of HT recipients mean that the electrophysiology of the transplanted heart and resultant arrhythmias are important causes of morbidity and mortality.
Pharmacology of the Transplanted Heart
The donor heart is distinct from the normal heart in its response to certain drugs. As discussed earlier, denervation means that normal autonomic regulation during exertion can be diminished or absent. This creates a dependence on circulating catecholamines to increase CO even with minimal stress. Cardiac myocytes shift from a predominance of type-1 to type-2 beta-adrenergic receptors. The most profound differences in the pharmacokinetics of the transplanted heart are unsurprisingly found with beta blockers and drugs targeting the autonomic nervous system.
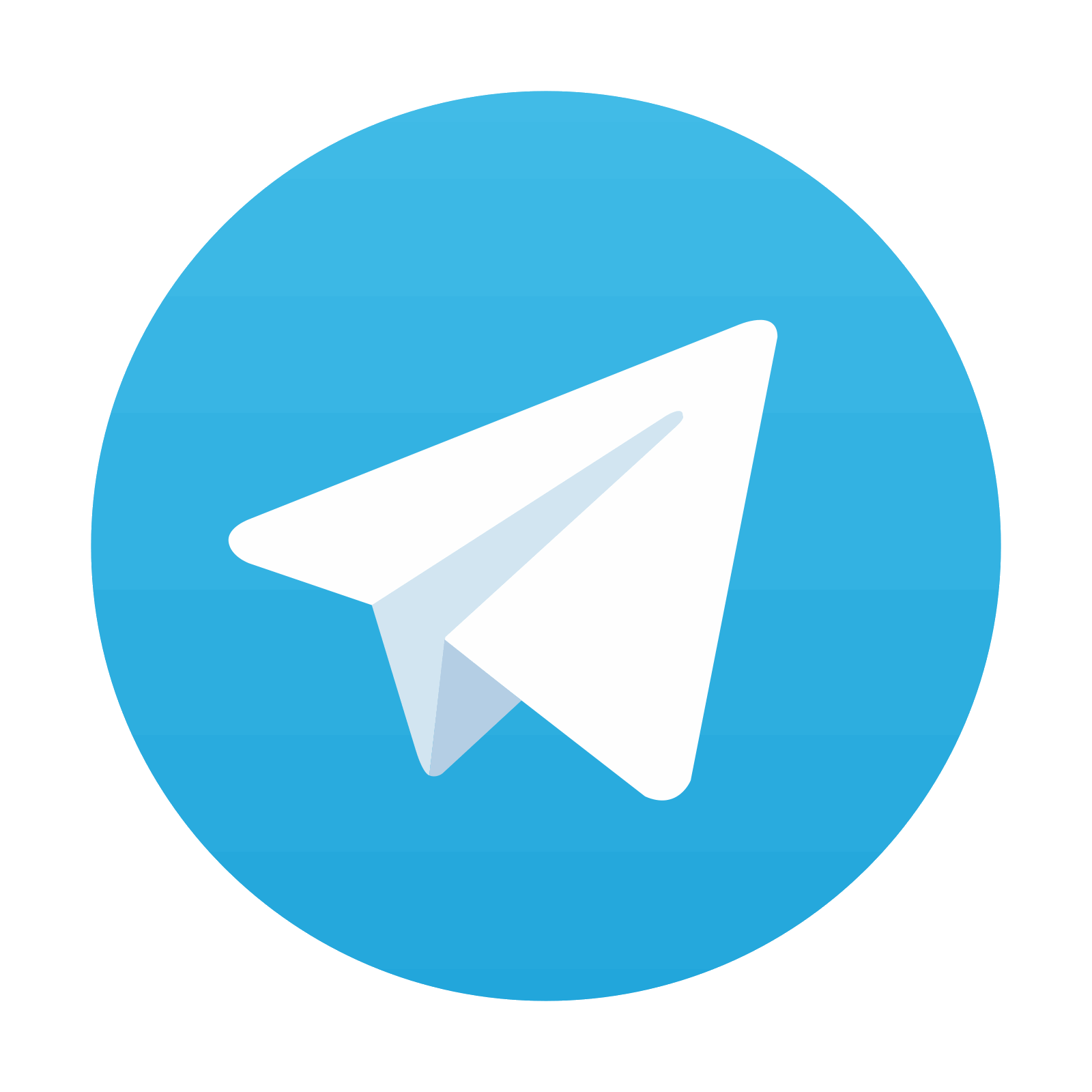
Stay updated, free articles. Join our Telegram channel
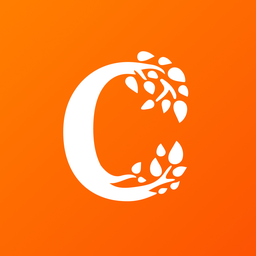
Full access? Get Clinical Tree
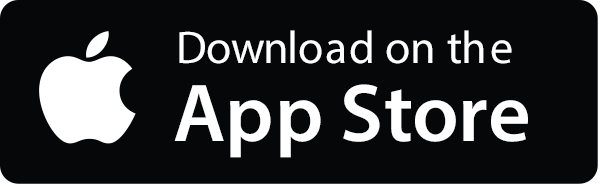
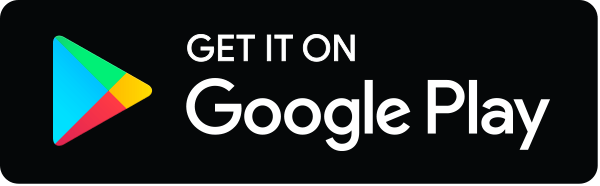