Fig. 2.1
Single beat method to measure right ventriculo-arterial coupling. Left: a maximum pressure (P max) is calculated from nonlinear extrapolation of early and late isovolumic portions of the right ventricular pressure (P RV) curve. A straight line is drawn from P max and the end-diastolic volume (EDV) tangent to the end-systolic pressure (P es)–volume (ESV) point. In the case of this theoretical illustration, RV maximum elastance (E max) coincides with end-systole, thus E max = (P max − P es)/SV, where SV is the stroke volume. The arterial elastance E a is defined by the ratio P es/SV
The single beat method can be applied with relative changes in volume measured by integration of the ejected flow rather than with measurements of absolute volumes. This is due to the fact that E max is not dependent on preload, or EDV [10]. An excellent agreement between directly measured P max (by clamping the main pulmonary artery for one beat) and calculated P max has been demonstrated in a large animal experimental preparation without pulmonary hypertension [18]. In states of increased impedance, lower vascular compliance, and increased pulsatile loading, a late systolic rise in RV pressure occurs. Therefore, it remains unknown if fitting a sine wave to the isovolumetric portions of the RV pressure tracing will accurately determine P max. Thus the single beat method to estimate RV-arterial coupling will require further validation in patients with severe pulmonary hypertension (Table 2.3).
Measurements of RV E max by conductance catheter technology and inferior vena cava balloon obstruction have been reported in normal volunteers [19, 20]. More recently, a limited number of E max determinations have been reported in patients with pulmonary arterial hypertension (PAH) either using the single beat approach, fluid-filled catheters, and magnetic resonance imaging (MRI) [21] or a multiple beat approach with venous return decreased by a Valsalva maneuver and conductance catheters [22]. The single beat approach with a high-fidelity Millar catheter and integration of a transonic measurement of pulmonary flow were reported in a patient with a systemic RV corrected and congenitally transposition of the great arteries [23]. These limited reports confirm the importance of systolic function adaptation to afterload previously demonstrated in various animal species [24], or experimental models of acute [24–26] or chronic [27–31] pulmonary hypertension.
Coupling of Systolic Function to Afterload
Measurements of systolic function are ideally load-independent, which means constancy over a wide range of immediate changes in preload or afterload. The requirement is met with an acceptable approximation by E max in intact hearts. This is because E max is the only point of the pressure–volume curve that is common in systole to ejecting and non-ejecting beats, and thus the optimal translation to a pressure–volume relationship of an isolated muscle active tension length relationship. However, as already discussed, E max adapts to afterload after a few beats. It is therefore important to correct E max for afterload.
There are three possible measurements of afterload [32, 33]. The first is maximum ventricular wall stress, which is approximated by the maximum value of the product of volume and pressure, divided by the wall thickness. This is a transposition of Laplace’s law for spherical structures, and thus problematic for the RV because of considerable regional variations of the internal radius. The second is based on measurements of the forces that oppose flow ejection, or the hydraulic load. This calculation optimally requires a spectral analysis arterial pressure and flow waves, with derived impedance calculations [33]. A more straightforward approach is to derive arterial elastance (E a) as it is “seen” by the ventricle and thus graphically determined using a pressure volume loop and by dividing maximal elastance pressure by SV (Fig. 2.1) [10].
Thus contractility coupled to afterload is defined by a ratio of E max to E a. The optimal mechanical coupling of E max to E a is equal to 1. The optimal energy transfer from the ventricle to the arterial system occurs at E max/E a ratios of 1.5–2 [10].
RV-Arterial Coupling in Experimental Pulmonary Hypertension
RV-arterial coupling measured with the E max/E a ratio has been investigated in various models of pulmonary hypertension. The results are summarized in Table 2.1. Acute hypoxia-induced increase in PVR was associated with a preserved RV-arterial coupling because of increased RV contractility [18, 24, 34–36]. Preserved RV-arterial coupling was also reported in pulmonary hypertension due to either microembolism or pulmonary arterial banding (PAB) [24]. Endotoxin shock was associated with a deterioration of RV-arterial coupling because of impaired contractility [26]. A chronic aorta-pulmonary shunt as a model of a persistent ductus arteriosus in growing piglets was associated with preserved RV-arterial coupling after 3 months [29, 37] but uncoupling occurred after 6 months because of decreased RV contractility resulting in a decreased cardiac output and increased right atrial pressure [30]. Persistent RV failure after tight transient PAB was characterized by a profound RV-arterial uncoupling because of a persistent decrease in contractility and reactive increase in PVR [38–41]. Monocrotaline-induced pulmonary hypertension was associated with RV-arterial uncoupling because of an insufficient increase in contractility to match increased afterload (Fig. 2.2) [31]. Mild pulmonary hypertension in pacing-induced heart failure was associated with RV-uncoupling due to the absence of an adaptive increase in RV contractility [42].


Fig. 2.2
Families of right ventricular pressure–volume loops at decreasing venous return in rats. E max is defined by a straight line tangent to the upper border of the pressure–volume loop families. Measurements are obtained in a control animal, after induction of pulmonary hypertension (PH) by the administration of monocrotaline and after induction of monocrotaline-PH under bisoprolol therapy. PH was associated with a marked adaptative increase in E max, which was further improved by bisoprolol therapy. From source data of Ref. [31]
Table 2.1
RV-arterial coupling in experimental models of pulmonary hypertension
Model | Animal | E max | E max/E a | References |
---|---|---|---|---|
Acute hypoxia | Dog, goat, pig | ↑ | – | |
Monocrotaline | Rat | ↑ | ↓ | [31] |
Sepsis, early | Pig | ↑ | – | [26] |
Sepsis, late | Pig | – | ↓ | [26] |
Acute embolism | Dog, goat, pig | ↑ | – | [24] |
Acute PA banding | Dog, goat, pig | ↑ | – | [24] |
AP shunting 3 mo | Pig | ↑ | – | [29] |
AP shunting 6 mo | Pig | ↓ | ↓ | [29] |
Acute RVF | Dog, pig | ↓ | ↓ | |
Chronic heart failure | Dog | – | ↓ | [42] |
Taken together, these studies support the notion of a predominant RV systolic function adaptation to increased afterload in various models of pulmonary hypertension, but with RV-arterial uncoupling in the context of inflammation (endotoxemia, monocrotaline), long-term increase in PVR, or left sided heart failure.
Pharmacology of RV-Arterial Coupling
In patients with severe pulmonary hypertension right ventricular function is a major determinant of quality of life, exercise capacity, and overall outcome [8]. Treatment strategies for these patients logically aim at the decrease in RV afterload often assessed by a measurement of PVR—or improvement in maximum cardiac output obtained by unloading the RV assessed by exercise capacity. However, it has been proposed that some of the vasodilators used for the treatment of PAH might also have intrinsic positive inotropic effects. There are data suggesting that this could be a mechanism of action of prostacyclins [43] or phosphodiesterase-5 inhibitors [44]. In addition to these perhaps “hidden” vasodilator drug actions, treatments specifically targeting the RV are now under consideration. The most obvious would be interventions aimed at the excessive neuro-humoral activation, which have been shown to improve survival in LV failure [45, 46]. Finally, patients with pulmonary hypertension may be exposed to the cardiovascular effects of general anesthesia or require treatments with inotropic drugs in case of severe RV failure [47, 48]. In all these circumstances, improved knowledge of the effects of the interventions on the components of RV-arterial coupling is desirable. Recent experimental animal studies reporting on the effects of pharmacological interventions on RV-arterial coupling are listed in Table 2.2.
Table 2.2
Effects of pharmacological intervention in experimental pulmonary hypertension (see Table 2.1)
Model | Drug | E max | E a | E max/E a | References |
---|---|---|---|---|---|
Acute hypoxia | Dobutamine | ↑ | – | ↑ | [18] |
Acute RHF | Dobutamine | ↑ | ↓ or – | ↑ | |
Acute RHF | Levosimendan | ↑ | ↓ | ↑ | |
Acute RHF | Norepinephrine | ↑ | – | [38] | |
Chronic heart failure | Milrinone | ↑ | – | ↑ | [42] |
Chronic heart failure | Nitroprusside | – | – | – | [42] |
Chronic heart failure | Nitric oxide | – | – | – | [42] |
Acute hypoxia | Propranolol | ↓ | ↑ | ↓ | [18] |
Monocrotaline | Bisoprolol | ↑ | – | ↑ | [31] |
AP shunting 3 mo | Epoprostenol | – | ↓ | ↑ | [37] |
Acute RHF | Epoprostenol | – | ↓ | ↑ | [40] |
Acute hypoxia | Sildenafil | – | ↓ | ↑ | [35] |
Monocrotaline | Sildenafil | ↑ | ↓ | ↑ | [49] |
Hypoxia | Isoflurane | ↓ | ↑ | ↓ | [29] |
Catecholamines may cause pulmonary vasoconstriction and tachycardia [47]. Moreover, treatment with catecholamine has been associated with increased mortality in patients with acute or chronic RV failure [48] and these data cause concern. Low-dose dobutamine increased RV-arterial coupling by an inotropic effect without [18, 39] or with [38] a decreased afterload. Low-dose norepinephrine improved RV-arterial coupling through an exclusive positive inotropic effect, which was however less pronounced than that achieved with low-dose dobutamine [38]. Experimentally acute administration of propranolol caused deterioration of RV-arterial coupling through combined negative inotrope effect and pulmonary vasoconstriction during acute hypoxia [18]. In the context of chronic administration of bisoprolol improved RV-arterial coupling by an improved contractility in monocrotaline-induced pulmonary hypertension (Fig. 2.2) [31].
Acute administration of epoprostenol or inhaled nitric oxide improved RV-arterial coupling exclusively via pulmonary vasodilation effects in a model of high-flow-induced pulmonary hypertension [37]. Acute epoprostenol partially restored RV-arterial coupling through an exclusive pulmonary vascular effect in PAB-induced persistent RV failure [40] or was associated with maintained RV-arterial coupling because of decreased contractility in proportion to a decreased PVR during acute hypoxia [36]. Levosimendan improved RV-arterial coupling through combined positive inotropy and vasodilation in PAB-induced persistent RV failure [39, 41]. Sildenafil improved RV-arterial coupling in hypoxia because of exclusive pulmonary vasodilation [35], but improved coupling by a positive inotropic effect in monocrotaline-induced pulmonary hypertension [49]. Bosentan had no intrinsic effect on contractility in pulmonary hypertension after 3 months of aorta-pulmonary shunting [29]. Milrinone improved RV-arterial coupling by an improved contractility in pacing-induced congestive heart failure with mild pulmonary hypertension, while nitroprusside or inhaled nitric oxide had no effect [42]. Isoflurane and enflurane caused deterioration of RV-arterial coupling because of a combined decrease in contractility and increase in PVR [34].
It is important to point out that acute and chronic effects of interventions on RV-arterial coupling in acute and chronic experimental pulmonary hypertension models may be quite different, as shown for β-blockers or sildenafil. This is a challenge to test of drugs in multiple experimental models and, makes the extrapolation to the clinical situation of patients with pulmonary hypertension difficult.
RV-Arterial Coupling Measurements in Patients with Pulmonary Hypertension
Measurements of both E max and E a have been reported in a small number of patients with pulmonary hypertension. In a first study of six patients with idiopathic PAH but no clinical RV failure, compared to six controls, Kuehne measured RV volumes by MRI and RV pressures using fluid-filled catheters, synchronized the signals and calculated E max and E a using the single beat method [21]. E max was increased threefold, from 1.1 ± 0.1 to 2.8 ± 0.5 mmHg/mL, but E a was increased from 0.6 ± 0.5 to 2.7 ± 0.2, so that the E max/E a ratio decreased from 1.9 ± 0.2 to 1.1 ± 0.1. Yet RV volumes were not increased, indicating “sufficient” coupling, at least under resting conditions.
Tedford reported on RV-arterial coupling in five patients with idiopathic PAH and seven with systemic sclerosis (SSc)-associated PAH [22]. In this study, RV volumes and pressures were measured with conductance catheters and E max defined by a family of pressure–volume loops as venous return decreased by a Valsalva maneuver (validated against inferior vena cava obstruction). Typical tracings are shown in Fig. 2.3. In IPAH patients, E max was 2.3 ± 1.1, E a 1.2 ± 0.5, and E max/E a preserved at 2.1 ± 1.0. In SSc-PAH patients, E max was decreased to 0.8 ± 0.3 in the presence of an E a at 0.9 ± 0.4, so that E max/E a was decreased to 1.0 ± 0.5. The authors also showed that there was no disproportionate decrease in pulmonary arterial compliance in SSc-PAH patients, suggesting that the depressed E max in SSc-PAH was not caused by a relatively higher pulsatile hydraulic load. Additionally, seven patients with SSc but without pulmonary hypertension maintained preserved coupling (E max/E a 2.3 ± 1.2).


Fig. 2.3
Right ventricular pressure–volume loops at decreasing venous return in a patient with systemic sclerosis (SSc)-associated pulmonary arterial hypertension (PAH), left, and in a patient with idiopathic PAH (IPAH), right. The slope of linearized maximum elastance pressure–volume relationship is higher at similar mean pulmonary artery pressure in IPAH. Source data from Ref. [22]
Finally, there is a case report of RV-arterial uncoupling in an asymptomatic young man with a congenitally corrected transposition of the great arteries [23]. In this patient, the systemic RV E max/E a ratio was of 1.2, in the range of the ratio reported in IPAH patients by Kuehne [21], while the pulmonary LV E max/E a was perfectly preserved at a value of 1.7. A decreased systemic RV E max/E a probably heralds failure, which is known to occur in these patients after several decades of life.
Together, these results confirm the dominant role of a homeometric adaptation of the RV to increased afterload, and document uncoupling when the hydraulic load remains too high for too long, or when systemic disease is present. From a methodological point of view, E max and E a show variability with a trend towards higher control values when measurements are based on families of pressure–volume loops rather than on single beat analysis. Targeted therapies in PAH patients might also have affected the results.
Thus it appears that in general RV-arterial coupling is maintained by an adaptive increase of the E max in PAH models of chronic hypoxia or aorta-pulmonary shunting when associated with only a moderate increase in pulmonary artery pressures. However, prolonged mechanical stress such as induced by 6 months of overcirculation in piglets, or due to altered LV function following several weeks of pacing in dogs, may cause uncoupling of the RV from the pulmonary circulation, increased filling pressures, and congestion. Monocrotaline has extra-pulmonary toxic effects and causes an inflammatory pulmonary vascular disease [50]. This is associated with decreased RV systolic function adaptation and leads to increased RV volumes. A general trend of reported studies is that pulmonary arterial obstruction such as pulmonary stenosis or PAB allows for a better and more prolonged preservation of RV-arterial coupling than the increased PVR of various forms of pulmonary vascular diseases [46, 51]. A heterometric adaptation may contribute to RV systolic function adaptation in any model depending on the volume status and the impact of an increased preload to afterload-induced changes, with volume overload as a cause of enhanced RV hypertrophy [52].
The determinants of long-term preservation of RV-arterial coupling in patients with severe pulmonary hypertension or with a systemic RV are not known. The molecular events leading to RV-arterial uncoupling and increased RV volumes remain to be identified. Knowledge of the signaling pathways responsible for maintained RV function in the presence of severely increased afterload may guide the development of new therapies [46].
The current understanding of the pathophysiology of RV failure include neuro-humoral activation, expression of inflammatory mediators, apoptosis, capillary loss, oxidative stress, and metabolic shifts, with variable fibrosis and hypertrophy [45, 46] (see Chap. 13). The exact sequence of events and interactions are being explored and has to be referenced to measurements of RV function, as illustrated in recent studies which showed inflammation and apoptosis correlated with decreased E max/E a in acute [53] as well as chronic [30, 54] models of RV failure.
Simplified Methods for the Measurement of RV-Arterial Coupling
Volume Measurements
A ratio of elastances can be simplified to a ratio of volumes, provided ESV is measured at the point of maximal elastance, not at the end of ejection. This is dependent on loading conditions. Pressure–volume relationships of the RV chronically exposed to increased pulmonary artery pressure tend to resemble LV pressure–volume loops, with a decreased difference between E max and Ees. LV pressure–volume loops after a Mustard procedure, which connects the LV to the pulmonary circulation are indistinguishable from the triangularly shaped normal RV, while the overall shape of the pressure–volume loop of the systemic RV resembles that of the normal LV [55].
Sanz measured ESV and SV by MRI and showed that the SV/ESV ratio is initially preserved in patients with mild pulmonary hypertension, but decreases with increasing disease severity [56]. One problem regarding the SV/ESV ratio is the inherent assumption that the ESP–ESV relationship is linear and that the line crosses the origin. This is incorrect, because ventricular volume at a zero filling pressure is positive. Therefore the ESP/ESV ratio under-estimates E max. There could be compensation by ESV being lower than the ventricular volume at the point of E max, but probably insufficiently so in pulmonary hypertension. Thus the SV/ESV as a simple volume measurement of RV-arterial coupling requires further evaluation and also of its functional and prognostic relevance. It can be reasoned that the SV/ESV ratio includes the information of RV ejection fraction (EF), or SV/EDV [32] in a less preload-dependent manner, but the validity of this remains to be established.
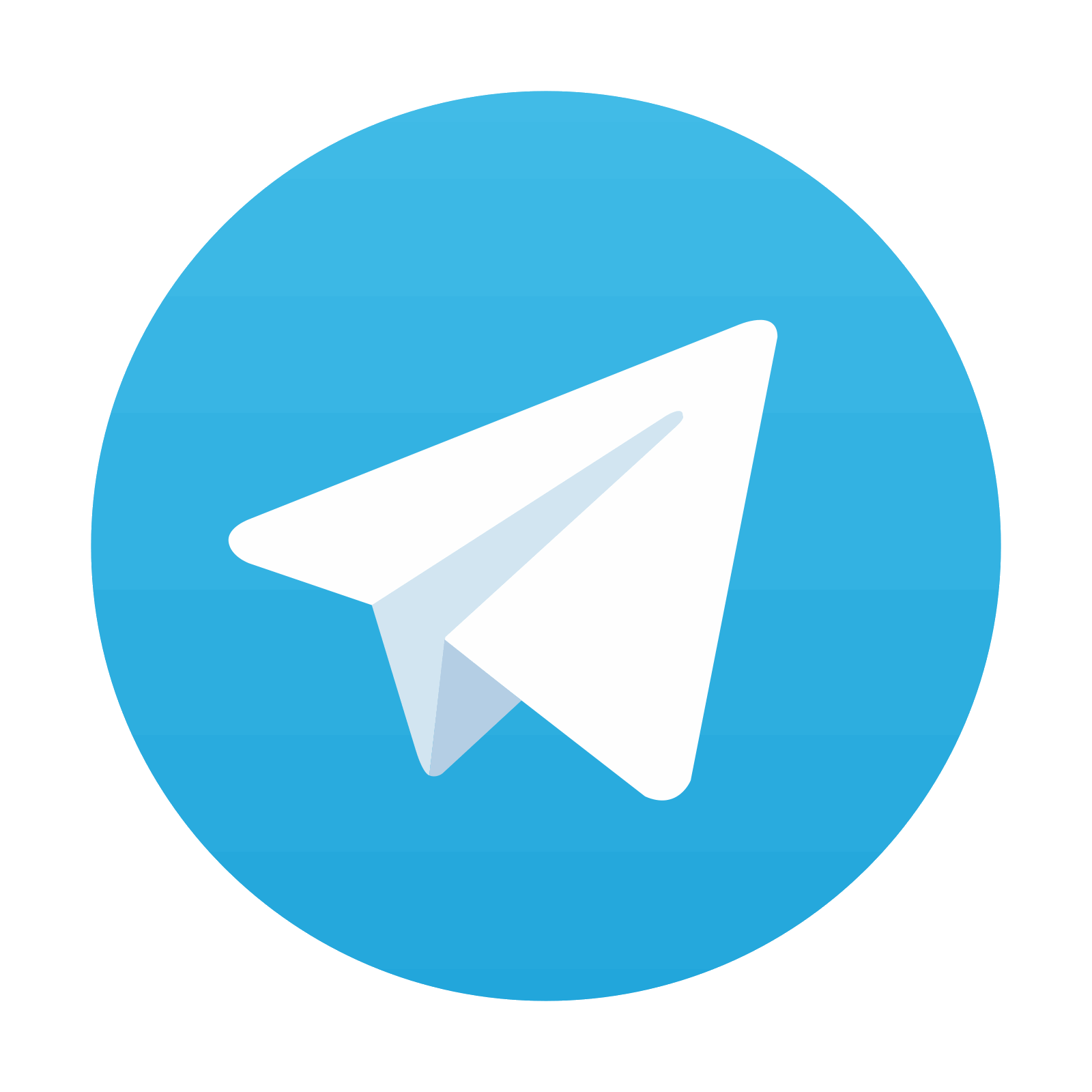
Stay updated, free articles. Join our Telegram channel
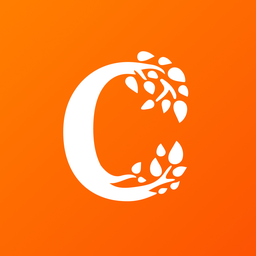
Full access? Get Clinical Tree
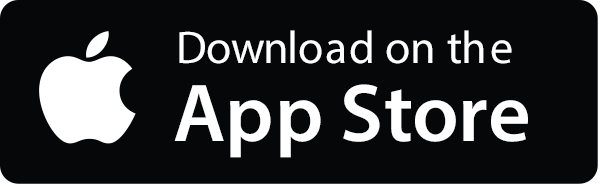
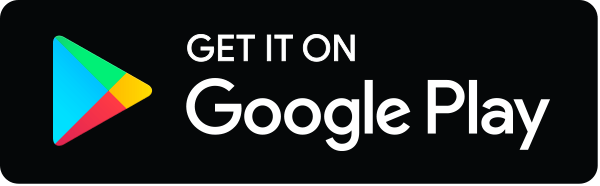