Physiology of the Pleural Space
The pleural space is the coupling system between the lung and the chest wall, and, accordingly, it is a crucial feature of the breathing apparatus. The pressure within the pleural space (the pleural pressure) is important in cardiopulmonary physiology because it is the pressure at the outer surface of the lung and the heart and the inner surface of the thoracic cavity. Because the lung, the heart, and the thoracic cavity are all distensible, and because the volume of a distensible object depends on the pressure difference between the inside and the outside of the object and its compliance, pleural pressure plays an important role in determining the volume of these three important structures.
PLEURAL PRESSURE
If the thorax is opened to atmospheric pressure, the lungs decrease in volume because of their elastic recoil, while at the same time, the thorax enlarges. With the thorax open, the volume of the thoracic cavity is approximately 55% of the vital capacity, whereas the volume of the lung is below its residual volume. With the chest closed and the patient relaxed, the respiratory system is at its functional residual capacity (FRC), which is approximately 35% of the total lung capacity (1). Thus, at FRC, the opposing elastic forces of the chest wall and lung produce a negative pressure between the visceral and the parietal pleura. This pressure, the pleural pressure, surrounds the lung and is the primary determinant of the volume of the lung. The pleural pressure represents the balance between the outward pull of the thoracic cavity and the inward pull of the lung (1).
Pleural Liquid Pressure versus Pleural Surface Pressure
There has been a controversy for many years as to whether there are two pleural pressures or one (2). The two different pressures had been proposed to explain a discrepancy obtained when the pleural pressure was measured in two different ways. If the pressure was measured using fluid-filled catheters, the vertical gradient obtained was approximately 1.0 cm H2O/cm vertical height. This pressure was designated the pleural liquid pressure and was believed to represent the pressure that influenced the absorption of fluid. If the pressure was measured using surface balloons or suction cups, then a gradient of 0.3 cm H2O/cm vertical height was obtained. This pressure was designated the pleural surface pressure and represented the balance between the outward pull of the thoracic cavity and the inward pull of the lung. It now appears that there is only one pressure, the pleural surface pressure, and that the discrepancies in the pressures arose because of the distortion from the catheters (3). It should be noted, however, that there is still a school of researchers who believe in the presence of two different pressures (4,5).
Measurement
Pleural pressure can be measured directly by inserting needles, trocars, catheters, or balloons into the pleural space. Direct measurement of the pleural pressure is not usually made because of the danger of producing a pneumothorax or of introducing infection into the pleural space. Rather, the pleural pressure is measured indirectly by a balloon positioned in the esophagus (6,7). Because the esophagus is a compliant structure
situated between the two pleural spaces, esophageal pressure measurements provide a close approximation of the pleural pressure at the level of the balloon in the thorax (7,8). Estimation of pleural pressure by means of an esophageal balloon is not without difficulties (8). The volume of air within the balloon must be small so that the balloon is not stretched and the esophageal walls are not displaced; otherwise, pleural pressure estimates are falsely elevated. Moreover, the balloon must be short and must be placed in the lower part of the esophagus. It has been demonstrated that reliable measurements of esophageal pressures can be made with micromanometers (9). The use of the micromanometer should circumvent some of the problems associated with esophageal balloons.
situated between the two pleural spaces, esophageal pressure measurements provide a close approximation of the pleural pressure at the level of the balloon in the thorax (7,8). Estimation of pleural pressure by means of an esophageal balloon is not without difficulties (8). The volume of air within the balloon must be small so that the balloon is not stretched and the esophageal walls are not displaced; otherwise, pleural pressure estimates are falsely elevated. Moreover, the balloon must be short and must be placed in the lower part of the esophagus. It has been demonstrated that reliable measurements of esophageal pressures can be made with micromanometers (9). The use of the micromanometer should circumvent some of the problems associated with esophageal balloons.
Gradients
Only one value for the pleural pressure is obtained when it is estimated by an esophageal catheter or balloon. It should be emphasized, however, that the pleural pressure is not uniform throughout the pleural space. A gradient in pleural pressure is seen between the superior and the inferior portions of the lung, with the pleural pressure being lowest or most negative in the superior portion and highest or least negative in the inferior portion (3). The main factors responsible for this pleural pressure gradient are probably gravity, mismatching of the shapes of the chest wall and lung, and the weight of the lungs and other intrathoracic structures (1).
The magnitude of the pleural pressure gradient appears to be approximately 0.30 cm H2O/cm vertical distance (3). It should be noted that over the last 30 years, there have been many studies directed at measuring the pleural pressure gradient and the resulting values have ranged from 0.20 to 0.93 cm H2O/cm vertical distance (3). The results have been largely dependent on the method used (3). It appears that the higher values were obtained with catheters that were large relative to the narrow pleural space and accordingly produced distortion of the pleura with subsequent alterations in the measured pressures (3).
In the upright position, the difference in the pleural pressure between the apex and the base of the lungs may be 8 cm H2O or more. Because the alveolar pressure is constant throughout the lungs, the end result of the gradient in the pleural pressure is that different parts of the lungs have different distending pressures. The pressure-volume curve is thought to be the same for all regions of the lungs; therefore, the pleural pressure gradient causes the alveoli in the superior parts of the lung to be larger than those in the inferior parts. The higher pressure gradient at the apex of the lung is thought to be responsible for the formation of pleural blebs almost exclusively at the apex of the lung. The pleural pressure gradients also account for some unevenness in the distribution of ventilation.
PLEURAL FLUID FORMATION
Fluid that enters the pleural space can originate in the pleural capillaries, the interstitial spaces of the lung, the intrathoracic lymphatics, the intrathoracic blood vessels, or the peritoneal cavity.
Pleural Capillaries
The movement of fluid between the pleural capillaries and the pleural space is believed to be governed by Starling’s law of transcapillary exchange (10). When this law is applied to the pleura as shown in Equation 2.1,

where [Q with dot above]r is the liquid movement; Lp is the filtration coefficient/unit area or the hydraulic water conductivity of the membrane; A is the surface area of the membrane; P and π are the hydrostatic and oncotic pressures, respectively, of the capillary (cap) and pleural (pl) space; and σd is the solute reflection coefficient for protein, a measure of the membrane’s ability to restrict the passage of large molecules (3). Widely varying values for σd have been reported. For example, the σd of the canine visceral pleura combined with the endothelium has been reported to exceed 0.80 (3), indicating a marked restriction in the movement of large molecules such as albumin. In contrast, the σd of the mediastinal pleura in the pig was reported to be between 0.02 and 0.05, indicating little restriction in the movement of large molecules (3). It appears that the restriction of protein by the pleural capillary endothelial-interstitial barrier is largely associated with the endothelium (3).
Estimates for the magnitude of the pressures affecting fluid movement from the capillaries to the pleural space in humans are shown in Figure 2.1. In the parietal pleura, a gradient for fluid formation is normally present. The hydrostatic pressure in the parietal pleura is approximately 30 cm H2O, whereas the pleural
pressure is approximately —5 cm H2O. The net hydrostatic pressure is therefore 30 – (-5) = 35 cm H2O, and this favors the movement of fluid from the capillaries in the parietal pleura to the pleural space. Opposing this hydrostatic pressure gradient is the oncotic pressure gradient. The oncotic pressure in the plasma is approximately 34 cm H2O. Normally, the small amount of pleural fluid contains a small amount of protein and has an oncotic pressure of approximately 5 cm H2O (11), yielding a net oncotic pressure gradient of 34 – 5 = 29 cm H2O. Thus, the net gradient is 35 – 29 = 6 cm H2O, favoring the movement of fluid from the capillaries in the parietal pleura to the pleural space.
pressure is approximately —5 cm H2O. The net hydrostatic pressure is therefore 30 – (-5) = 35 cm H2O, and this favors the movement of fluid from the capillaries in the parietal pleura to the pleural space. Opposing this hydrostatic pressure gradient is the oncotic pressure gradient. The oncotic pressure in the plasma is approximately 34 cm H2O. Normally, the small amount of pleural fluid contains a small amount of protein and has an oncotic pressure of approximately 5 cm H2O (11), yielding a net oncotic pressure gradient of 34 – 5 = 29 cm H2O. Thus, the net gradient is 35 – 29 = 6 cm H2O, favoring the movement of fluid from the capillaries in the parietal pleura to the pleural space.
![]() FIGURE 2.1 ▪ Various pressures that normally influence the movement of fluid in and out of the pleural space in species with a thick visceral pleura, such as humans. |
The net gradient for fluid movement across the visceral pleura in humans is probably close to zero, but this has not been demonstrated (Fig. 2.1). The pressure in the visceral pleural capillaries is approximately 6 cm H2O less than that in the parietal pleural capillaries because the visceral pleural capillaries drain into the pulmonary veins. Because this is the only pressure that differs from those affecting fluid movement across the parietal pleura and because the net gradient for the parietal pleura is 6 cm H2O, it follows that the net gradient for fluid movement across the visceral pleura is approximately zero. It is also likely that the filtration coefficient (Lp) for the visceral pleura is substantially less than that for the parietal pleura because the capillaries in the visceral pleura are much farther from the pleural space than those in the parietal pleura (12).
![]() FIGURE 2.2 ▪ Various pressures that normally influence the movement of fluid in and out of the pleural space in species with a thin visceral pleura, such as the dog. See text for explanation. |
The movement of pleural fluid is not the same across all the parietal pleura. Wang and Lai-Fook (13) used Evans blue-dyed albumin to study regional pleural filtration of prone anesthetized rabbits. They reported that there appeared to be more fluid formation across the parietal pleura over the ribs compared with the intercostal spaces. In contrast, pleural liquid absorption was primarily in the parietal pleura adjacent to the intercostal space rather than in the parietal pleura overlying the ribs. There was also more fluid formation over the caudal ribs than over the cranial ribs (13). If the breathing frequency was increased, more fluid was formed (13).
The transpleural exchange of fluid is species dependent. Humans and sheep have a thick visceral pleura and its blood supply is from the bronchial artery rather than from the pulmonary artery (14). However, many species, such as the rabbit and the dog, have a thin visceral pleura that receives its blood supply from the pulmonary circulation. In such a situation, as shown in Figure 2.2, the net gradients favor pleural fluid formation across the parietal pleura and pleural fluid absorption through the visceral pleura.
Interstitial Origin
The origin of much of the fluid that enters the pleural space in disease states is the interstitial spaces of the lungs. Either high-pressure or high-permeability pulmonary edema can lead to the accumulation of pleural fluid. When sheep are volume overloaded to
produce high-pressure pulmonary edema, approximately 25% of all the fluid that enters the interstitial spaces of the lungs is cleared from the lung through the pleural space (15). Within 2 hours of starting the volume overloading, the amount of fluid entering the pleural space increases, and within 3 hours, the protein concentration in the pleural fluid is the same as that in the interstitial spaces of the lungs (15). The amount of pleural fluid formed is directly related to the elevation in the wedge pressure. Increases in pleural fluid accumulation occur only after the development of pulmonary edema (16).
produce high-pressure pulmonary edema, approximately 25% of all the fluid that enters the interstitial spaces of the lungs is cleared from the lung through the pleural space (15). Within 2 hours of starting the volume overloading, the amount of fluid entering the pleural space increases, and within 3 hours, the protein concentration in the pleural fluid is the same as that in the interstitial spaces of the lungs (15). The amount of pleural fluid formed is directly related to the elevation in the wedge pressure. Increases in pleural fluid accumulation occur only after the development of pulmonary edema (16).
The pulmonary interstitial space is the predominant origin of pleural fluid in patients with congestive heart failure. The likelihood of a pleural effusion increases as the severity of pulmonary edema increases (17). In addition, the presence of pleural effusions is more closely correlated with the pulmonary venous pressure than with the systemic venous pressure (17). However, patients with right heart failure due to pulmonary hypertension may have pleural effusions although their wedge pressures are normal (18). The origin of the pleural fluid in this situation is probably the capillaries in the parietal pleura (18). The amount of fluid that enters the pleural space is also increased when there is increased interstitial fluid due to high-permeability pulmonary edema. When increased-permeability edema was induced in sheep by the infusion of oleic acid, again, pleural fluid accumulated only after pulmonary edema developed (19). In this study, there was no morphologic evidence of pleural injury. When pulmonary edema is induced by xylazine (20) or hyperoxia (21) in rats, or by ethchlorvynol in sheep (22), the high-protein pleural fluid appears to originate in the interstitial spaces of the lungs. The pleural fluid associated with experimental Pseudomonas pneumonia in rabbits originates in the lung (23). It is likely that the origin of the pleural fluid with many conditions associated with lung injury, such as pulmonary embolization and lung transplantation, is also the interstitial spaces of the lung (2). In experimental studies of hydrostatic and increased-permeability edema, a pleural effusion develops when the extravascular lung water has reached a critical level in a certain amount of time (24). The necessary level of edema appears to be between 5 and 8 g of fluid/gram of dry lung, depending on whether the edema is secondary to hydrostatic edema, oleic acid lung injury, or α-naphthyl thiourea lung injury (24). With increasing levels of interstitial fluid, it has been shown that the subpleural interstitial pressure increases (25). The barrier to the movement of fluid across the visceral pleura appears to be weak, even though the visceral pleura is thick (26). Therefore, once the subpleural interstitial pressure increases, it follows that fluid will traverse the visceral pleura to the pleural space.
Peritoneal Cavity
Pleural fluid accumulation can occur if there is free fluid in the peritoneal cavity and if there are openings in the diaphragm. Under these conditions, the fluid will flow from the peritoneal space to the pleural space because the pressure in the pleural cavity is less than the pressure in the peritoneal cavity. The peritoneal cavity is the origin of the pleural fluid in hepatic hydrothorax (Chapter 9), Meigs’ syndrome (Chapter 20), and peritoneal dialysis (Chapter 9) (27). There are no direct lymphatic connections between the peritoneal and pleural cavities (28).
Thoracic Duct or Blood Vessel Disruption
If the thoracic duct is disrupted, lymph will accumulate in the pleural space, producing a chylothorax (see Chapter 26). The rate of fluid accumulation with chylothorax can be more than 1,000 mL/day. When the thoracic duct is lacerated in dogs, sizeable pleural effusions begin to develop almost immediately (29). In a like manner, when a large blood vessel in the thorax is disrupted owing to trauma or disease, blood can accumulate rapidly in the pleural space, producing a hemothorax (see Chapter 25).
Origin of Normal Pleural Fluid
It is believed that the fluid that normally enters the pleural space originates in the capillaries in the parietal pleura (30). The normal pleural fluid production is approximately 0.01 mL/kg/hour in awake sheep and 0.02 mL/kg/hour in rabbits (30). If these rates are extrapolated to human beings, the amount of pleural fluid formed daily in a 50-kg individual would be approximately 15 mL (30). The origin of the fluid does not appear to be the interstitial spaces of the lung because the protein level in the interstitial spaces is normally approximately 4.5 g/dL, whereas the protein level in normal pleural fluid is only approximately 1 to 1.5 g/dL. From Figure 2.1, it appears unlikely that the fluid originates from the visceral pleura. Likewise, both a lymphatic origin and a peritoneal cavity origin appear unlikely. Supporting evidence for this theory has been provided by Broaddus et al. (31).
These workers measured the vascular pressures and the pleural fluid protein levels in sheep of different ages. They found that the systemic vascular pressures progressively increased with age, whereas the pleural fluid protein levels progressively decreased with age. These findings support a parietal pleural origin for normal pleural fluid because higher vascular pressures should produce pleural fluid with lower protein levels (31). Studies in rabbits with Evans blue-dyed albumin have demonstrated that most fluid originates in the parietal pleura over the ribs (13).
These workers measured the vascular pressures and the pleural fluid protein levels in sheep of different ages. They found that the systemic vascular pressures progressively increased with age, whereas the pleural fluid protein levels progressively decreased with age. These findings support a parietal pleural origin for normal pleural fluid because higher vascular pressures should produce pleural fluid with lower protein levels (31). Studies in rabbits with Evans blue-dyed albumin have demonstrated that most fluid originates in the parietal pleura over the ribs (13).
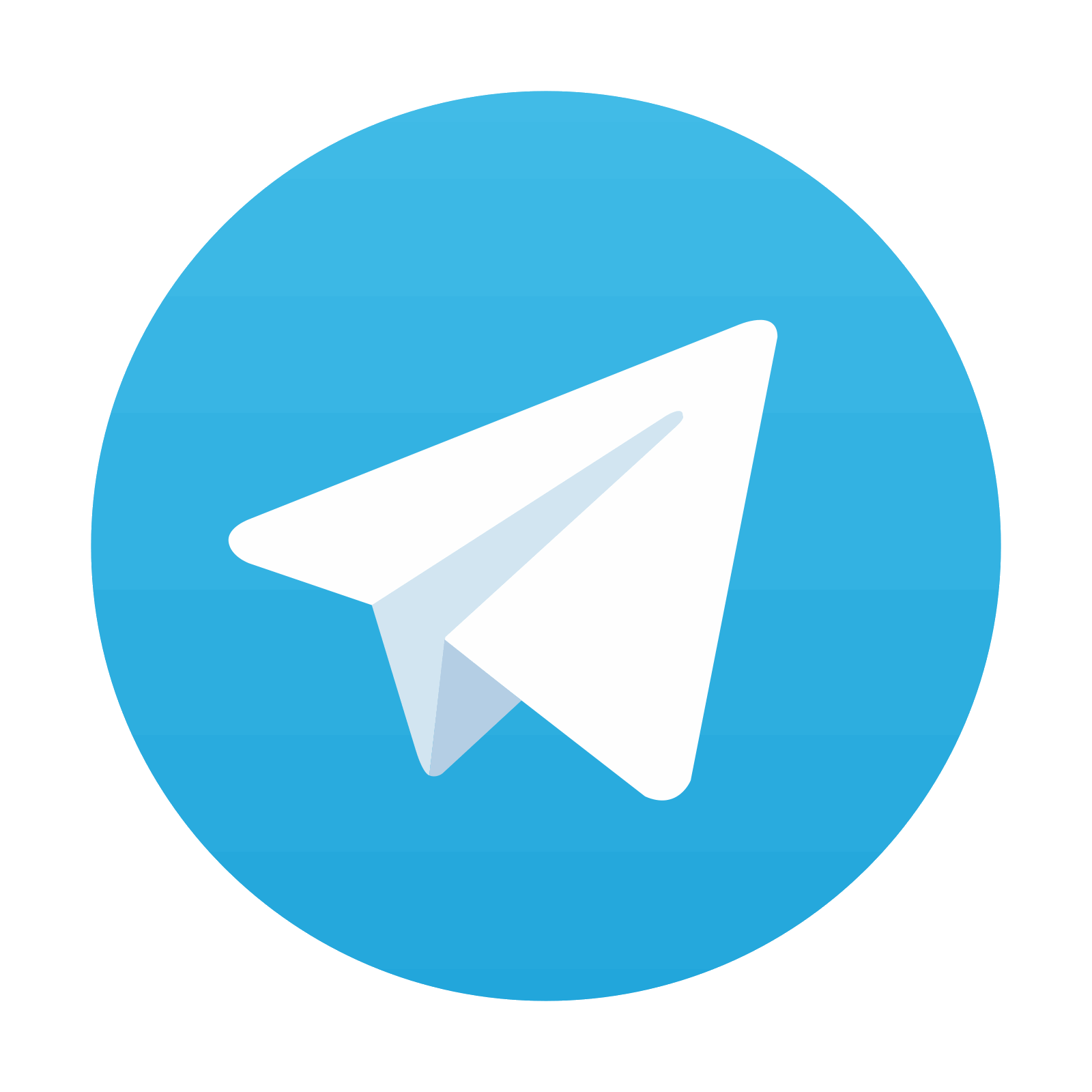
Stay updated, free articles. Join our Telegram channel
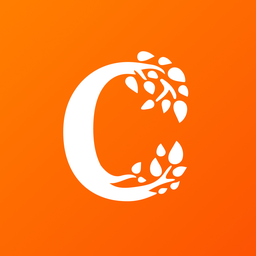
Full access? Get Clinical Tree
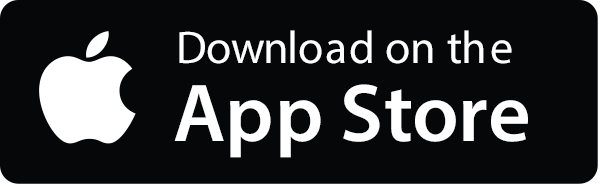
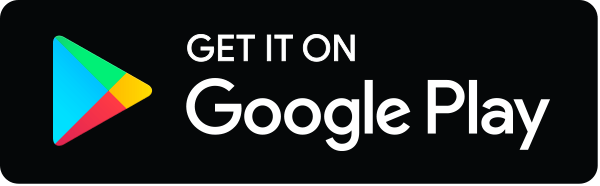