Fig. 30.1
Stem and progenitor cells defined by proliferative and differentiation capacity. This figure depicts the reduction in proliferative potential and differentiation potential during cell maturation. It should be kept in mind that these differences are not absolute. Some mature cells, e.g. lymphocytes, can proliferate extensively, and some mature cells retain the potential to differentiate further. B cell class switching would be an example
Self-renewal in its strictest sense implies unlimited proliferative capacity, since the daughters are identical to the parent cell. This is impossible to verify experimentally, at least without unlimited funding and time. A more practical definition would be the ability to function and produce cells beyond the normal lifespan of the organism. E.g. murine hematopoietic stem cells can be transplanted serially, and repopulate new hosts, at least five times [9–11]. While this is not unlimited, it clearly suffices for the animal, even if the need for cells is higher than normal, such as following (repeated) serious injury and blood loss. In addition, it is important to note that the proliferative limitations encountered may well be a result of the experimental conditions (repeated transplantation into an irradiated environment) and do not necessarily represent the true limitations inherent to the stem cells themselves.
Cells that come much closer to demonstrating unlimited proliferative capacity are cells that can be maintained in culture, such as embryonic stem cells. Many of the murine ES cell lines have been in culture now for over 20 years, and have been used and expanded extensively by many different laboratories, without losing the ability to act as pluripotent stem cells with the capacity to regenerate a mouse.
The differentiation capacity is the main distinguishing feature between different types of stem cells. Pluripotent stem cells such as embryonic stem cells and iPS cells have the ability to generate all the different cells in the body (and can generate the organism as a whole). These cells represent germ line stem cells, the cells that allow life to pass from one generation to the next. Other types of stem cells in the adult body (sometimes referred to as “adult stem cells”) are more restricted; they can differentiate into many (multipotent) or a few (oligopotent) cell lineages within the germ layer from which they are derived. Mesenchymal stem cells and hematopoietic stem cells, for instance, can make subsets of mature mesoderm cells. The ability of stem cells to produce cells derived from other germ layers was hotly debated a decade ago, a debate that was referred to as the stem cell plasticity debate. It was claimed, for instance, that blood cells could differentiate into brain cells, or liver cells [12, 13]. More extensive analysis revealed, however, that much of this apparent plasticity was due to experimental artifacts, such as cell fusion. Plasticity or transdifferentiation–differentiation into a lineage that is not part of the normal repertoire of the stem cell, seems to happen rarely, if at all, under normal conditions [14–16]. Interestingly, what has surfaced since this debate is the ability to reprogram cells, even regular somatic cells without stem cell ability, into pluripotent stem cells (the so-called iPS cells) with the ability to produce cells from endodermal, mesodermal and ectodermal origin [17–21].
Once stem cells commit to differentiation, and leave the stem cell pool, they typically go through an intermediate stage often referred to as progenitors (Fig. 30.2). In some systems other names are used for these cells: In skin, for instance, they are called transit-amplifying cells. Practically, the difference between stem and progenitor cells can be hard to make. Progenitor cells may still have the potential to generate several different types of mature cells. Progenitor cells often have extensive proliferative capacity, and much of the expansion needed to amplify the offspring of the rare stem cells into the many mature cells needed in tissues such as skin, blood and gut epithelium, occurs at the progenitor level. However, progenitor cells do not self-renew. With every cell division they move closer to the mature state. New cells from any given progenitor cell are only generated for a relatively brief period of time, whereas stem cells may produce new cells throughout life. However, the distinction between newly generated and older pre-existing cells can be hard to make when the mature cells are long-lived.


Fig. 30.2
Classical stem cell differentiation pattern. This would apply to hematopoietic cell differentiation, but also to other types of cells, e.g. skin [22]. Maintenance of stem cells is assured by stem cell self-renewal. Much of the proliferation needed to obtain the required number of mature cells occurs at the progenitor level. Progenitors can initially be oligopotent (more than one cell fate possible) or can be committed to a single outcome. Full commitment of progenitors to a new more restricted stage can take several days. Mature cells, depending on type, may retain the capacity for extensive proliferation (e.g. lymphocytes) or may not be able to divide at all (red blood cells, muscle cells)
Diagrams, such as the tree shown in Fig. 30.2 often depict progenitors as very distinct entities, with differentiation steps taking them from more primitive to less primitive, more restricted, progenitors, eventually resulting in mature cells. These entities are based on purification methods such as Fluorescence Activated Cell Sorting (FACS) or assays such as colony assays. The ability to purify, study and use progenitors with specific potential is very useful and informative. The cells themselves, however, can be thought of as being on a continuum, gradually shifting from one phenotype to the next, gradually restricting their differentiation potential.
Stem Cells: Niche and Regulation
Obviously, in view of their proliferative potential, stem cells need extensive regulation. While some stem cells, such as neuronal stem cells, are mostly quiescent and generate few offspring under normal conditions, other stem cells have to continuously generate large numbers of mature cells. For example, in the hematopoietic system more than 1011 mature cells need to be produced every day [23–25]. To control this expansion, and prevent it from escaping control, stem cells typically need specific signals to maintain their stem cell potential. In the absence of these signals the cells either differentiate along a default pathway, or undergo apoptosis. Yet it is also essential that the stem cell numbers are maintained. Loss of e.g. colon or hematopoietic stem cells would result in death in days to weeks. Stem cell homeostasis (Fig. 30.3) requires that under steady state conditions, following stem cell division, on average one of the daughter cells remains a stem cell, while the other cell either commits to differentiation, or undergoes apoptosis. The place where stem cells receive these signals is often referred to as the stem cell niche (Fig. 30.4). This can be a very defined, physical environment. Figure 30.4 shows two examples of stem cell niches. In the Drosophila spermatogonium the location of the stem cells that produce the spermatozoa is exactly defined. They are at the tip of the gonad, in direct contact with other cells known as hub-cells. These hub cells provide the signals necessary for the stem cells to remain stem cells. Once the stem cells divide the cell division is oriented such that one daughter cell remains in contact with the hub cells, and the other daughter loses contact. The daughter cell that has lost contact commits to differentiation, resulting in an obligatory asymmetric cell division. A similar asymmetric division occurs for the accompanying cyst cells that surround the germ cell throughout the differentiation process. The critical signal, through the Jak/Stat pathway, provided by the hub cells is called Unpaired (Upd), which is a ligand for the receptor Domeless (Dome) and the associated Jak kinase Hopscotch (Hop). Upon binding of Upd the receptor is phosphorylated by Hop, followed by Stat binding, phosphorylation, dimerization, and translocation to the nucleus. Mutants in which the cyst cells overexpress the ligand Upd never form functional sperm. Instead the spermatogonium fills up with stem cells. Mutants without functional Stat do not maintain stem cells, but have a single early wave of spermatogenesis [26, 27].



Fig. 30.3
Stem cell homeostasis. A stem cell pool size is regulated at different levels. Symmetrical self-renewing divisions (both daughters remain stem cells) are the main mechanism for expansion, while differentiation and apoptosis are the main exits from the stem cell pool. Migration, resulting in either resettlement in a stem cell niche, or in exit from the stem cell pool through differentiation or apoptosis, can complicate this process

Fig. 30.4
The concept of the stem cell niche. Shown are two examples of stem cell niches, the Drosophila spermatogonium, and the mammalian hematopoietic stem cell. (a) In this niche concept the germ and somatic cells in the apical tip of the testis that are in direct contact with the hub cells remain stem cells. Once this contact is lost, through oriented cell division, the cells start their differentiation toward spermatozoa, which takes many steps and several more cell division. (b) In the hematopoietic system the organization is more flexible in that HSC have the ability to move from their resting niches near the bone to the vasculature, and back. Both environments differ significantly, e.g. in O2 levels. Most differentiation (including the accompanying proliferation) also takes place in the bone marrow. The resulting mature cells enter the blood to leave the bone marrow
In the mammalian bone marrow the location of the hematopoietic stem cells has been less clear. One reason is that bone marrow is typically studied as a cell suspension by flow cytometry and colony- or reconstitution assays after it has been removed from the marrow spaces, rather than by histology in situ. Also, regulation is more complex in that hematopoietic stem cells can leave their niches in the bone marrow and travel through the vasculature to new locations such as spleen and liver. They do so in a regulated fashion several times during development [28], but retain the ability to migrate in adult life as a response to various stimuli [29, 30], with CXCR4/SDF-1α signaling as a central component. Clinically, this is used to harvest them: Rather than harvest bone marrow directly hematopoietic stem cells are harvested as Mobilized Peripheral Blood (MPB) by leukapheresis after treatment of the donor with the growth factor G-CSF and the chemotherapeutic cyclophosphamide. Nevertheless, much has been learned in recent years about the important cells in the niche, and the signals governing stem cell behavior. An in-depth discussion is outside of the scope of this more general chapter, and many reviews can be used as starting points to further probe the literature, see e.g. [31–36]. A variety of cells have been reported to be part of this niche, including osteoblasts [37–39], sinusoidal endothelium [40], CXCL12 expressing reticular cells [41], adipocytes [42] and mesenchymal stem cells [43]. A number of regulators have been postulated to be involved in stem cell maintenance in this niche. These include Wnt proteins [44–47], the Notch pathway [48–50], insulin-like growth factor (IGF-2) and angiopoietin-like proteins [51–53]. Important regulators of cell cycle progression include the p16Ink4a–CDk4/6Rb and p19Arf-P53-P21Cip1 pathways [54, 55]. Another important factor for stem cells is the ability to maintain telomere length during successive cell divisions. To accomplish this stem cells express telomerase. Loss of telomerase can limit stem cell self-renewal during serial transplantation [56] and aging [57]. It has recently been reported that telomerase activity is regulated by Wnt/β-catenin in stem cells [58]. Despite all of the progress that has been made in characterizing the regulation of hematopoietic stem cells no clear conditions have been defined yet that allow for robust expansion of these cells outside of the body [59], something that would be extremely useful in broadening their therapeutic potential. This indicates that our understanding of HSC self-renewal, and the molecular players involved, is still lacking.
Other types of stem cells, in particular Embryonic Stem cells, are capable of extensive expansion outside of the body using defined conditions without reduction of their pluripotency, their ability to differentiate into all tissues of the body. Much has been learned about the factors governing unlimited self-renewal and developmental potential [55, 60–62]. Central components include the transcription factors Oct4, Sox2 and Nanog. These transcription factors, which interact physically, form a finely tuned network, disturbance of which can lead to loss of pluripotency. Additional proteins, like the zinc-finger DNA binding protein Ronin, are also involved and can prevent differentiation [63]. In addition there is regulation at the epigenetic level, with Polycomp complexes playing an important role [62]. Ultimately demonstrating the importance of these pathways has been the ability to use forced expression of several of these proteins to convert somatic cells into pluripotent stem cells [61] (See iPSC section below.)
Overall, these examples illustrate that niches are important and specific, and their presence can limit and regulate stem cell presence. This regulation is especially critical for cells that continuously produce large numbers of mature cells. Loss of control of expansion would quickly result in a proliferative disease, or even cancer.
Stem Cells: Clinical Use
The specifics of the use of stem cells in the heart are discussed elsewhere in this book. This section will be limited to a more general overview of the current and potential use of stem cells in medicine. As mentioned earlier, hematopoietic stem cell transplantation is currently in widespread use, albeit that this involves transfer of stem cell containing cell preparations, and not highly purified HSC. This is an important distinction, as the main indication for use is the treatment of malignancies. Autologous transplants in which patients receive their own cells, harvested prior to intensive chemotherapy, thus carry a risk of reseeding the patient with cancer cells that contaminate the graft. Small-scale studies with highly purified autologous hematopoietic stem cells indicate that high level purification indeed improves outcome [64, 65]. Allogeneic transplants, in which the patient receives cells from a different individual, do not carry this risk, but, specific for hematopoietic transplants, may result in adaptive immune cells in the graft reacting against the host body. This is known as graft-versus host disease, and is a potentially lethal complication of allogeneic hematopoietic cell transplantation [66–68].
Hematopoietic stem cells have the potential, and are starting to be used for many other therapies [69], including the treatment of autoimmune diseases [70], the treatment of inherited metabolic diseases [71] and the induction of tolerance for solid organ transplantation [69, 72–74].
Skin is another tissue that is transplanted in routine clinical use, and that depends for functioning on the transplanted stem cells [75–77]. Skin, like blood, is continuously regenerated from stem cells. Old cells slough off and are discarded. Autologous transplants, in which skin is harvested at one place of the body and transplanted to another, e.g. to cover a burn, results in lasting engraftment. Allogeneic skin transplants, typically harvested from a deceased donor, are used as temporary cover, as they will be rejected by the immune system.
Other types of stem cells, especially mesenchymal stem cells, are being tested in clinical trials [78–80], including trials addressing cardiac failures [81–83], but are not yet in routine use. Mesenchymal stem cells can be harvested relatively easily from various sources, including bone marrow and adipose tissue, expanded in culture and can differentiate into many tissues, including bone, adipose, myogenic and hepatic cells [84]. In addition, mesenchymal stem cells can modulate immune responses [78, 80]. Neuronal stem cells are also being tested in initial clinical trials, e.g. [85–87]. Other cells, including ES cells [88, 89] and iPS cells [21, 90–93], are being developed for eventual clinical use.
Stem Cells and Cancer
Stem cells, through their ability to persist for long periods of time while cycling, even if slowly, are prime candidates to collect the mutations necessary to allow escape from their normal controls and become transformed. Like normal, non-transformed, stem cells cancer stem cells may remain dependent on a stem cell niche for some of their regulatory (pro-mitogenic) signals [35, 94], even though they may have lost other parts of the normal regulatory circuitry, like essential tumor suppressor genes that should prevent these cells from proliferating [55, 95].
Interestingly, it has been recognized recently that cancers themselves tend to be organized in cancer stem cells, which may only make up a small part of the tumor and the bulk of the tumor cells which are derived from these stem cells, analogous to differentiated cells in normal tissues [96–101]. In this model the cancer stem cells are both necessary to maintain the tumor, and sufficient, if not eradicated completely, to regrow the tumor (relapse) after treatment.
Induced Pluripotent Stem Cells (IPSC)
One of the most exciting developments in stem cell biology in the last decade has been the discovery that a stem cell phenotype, even that of pluripotent stem cells, can be induced in somatic cells following transduction with a limited set of genes. In the initial landmark studies mouse fibroblasts, transduced with Oct4, Sox2, Klf4 and c-Myc, were reprogrammed into cells very similar to Embryonic Stem cells [19]. These results were rapidly confirmed with human cells, and a slightly different gene combination (Oct4, Sox2, Nanog and Lin28) [18, 20]. Many different types of cells are open to reprogramming, including fibroblasts (above), keratinocytes [102], neural stem cells, hepatocytes and gastric epithelial cells [103], adipocytes and hematopoietic cells [104].
IPS cells have a number of obvious advantages over ES cells. Unlike ES cells, their origin is not ethically controversial. Furthermore, the ability to generate pluripotent cells from any donor holds great promises for the development of specific disease models. However, it remains to be established how closely iPS cells resemble ES cells, and the presence, at least in the initial experiments, of the oncogene c-Myc in the transformation mix gives pause when contemplating clinical use [61]. The optimal gene combination for induction of iPS cells remains a subject of interest [61], complicated by the more recent observations that show that it is possible to reprogram cells to a different fate without passing through the pluripotent intermediate stage [105, 106]. It is possible, e.g. to reprogram fibroblasts directly into neurons [107] or cardiomyocytes [108]. While in early stages, this clearly further increases the possibilities for creating genetically matched tissues for research and therapy.
Stem Cells and the Heart
When discussing stem cells in the context of the heart in general, or heart failure in particular, there are several different points of view that can be considered. Stem cells play a role in generating the structures of the heart [109]. Heart specification starts with the so-called first heart field, which will initially form a tube with endocardial layer on the inside, and a myocardial layer on the outside. Through differential growth and folding this will eventually result in a multi-chambered heart, with cells from the first heart field forming the left side of the heart, while cells from a second heart field mostly form the right side and outflow tract [110, 111]. To some extent, progenitors remain present once the heart is formed and provide a certain level of regenerative potential. There may be other stem cells as well that can be used to ameliorate the function of damaged heart tissue [112–114]. In addition to endogenous heart cells [115], there are other somatic stem cells (like mesenchymal stem cells) [116] and pluripotent stem cells (ES cells or iPS cells) [21, 110]. The therapeutic use of stem cells for cardiac repair is discussed in more detail elsewhere in this book.
Interestingly, while regeneration of functional myocardial tissue is limited in heart failure following myocardial infarct or other damage, cardiac progenitor cells are present in the myocardium, e.g. [117–119]. It may be possible to use these cells to improve function in failing hearts. In addition to the presence of cardiac progenitors, it has recently also been shown that at least some existing cardiomyocytes retain the potential to divide and replace cells [120]. This potential of cardiomyocyte proliferation can be greatly stimulated with exogenous administration of select micro RNA’s (miRNA’s) [121].
Conclusion
Stem cells play an essential role, during development and continuing in adult life. While much has been learned about the biology of stem cells in different organs and organisms, much more remains to be learned. The clinical promise is enormous, and current use barely scratches the surface. To develop this promise into eventual clinical reality it will be essential to follow the winding path, despite its frequent switchbacks, carefully. As tempting as shortcuts can be, in systems as complex as those discussed here, a methodical approach is the only reliable method of distinguishing what does and does not work when translated to the clinic. The biggest danger in attempting and failing at shortcuts to the clinic is establishing as common knowledge that something “does not work” before it has been tested. This can prevent promising avenues from being explored and developed.
References
1.
Jacobsen LO, Marks EK, Robson MJ, Gaston EO, Zirkle RE. Effect of spleen protection on mortality following x-irradiation. J Lab Clin Med. 1949;34:1538.
2.
Lorenz E, Uphoff D, Reid TR, Shelton E. Modification of irradiation injury in mice and guinea pigs by bone marrow injections. J Natl Cancer Inst. 1951;12:197–201.PubMed
3.
Becker AJ, Mc CE, Till JE. Cytological demonstration of the clonal nature of spleen colonies derived from transplanted mouse marrow cells. Nature. 1963;197:452–4.PubMed
4.
Siminovitch L, McCulloch EA, Till JE. The distribution of colony-forming cells among spleen colonies. J Cell Physiol. 1963;62:327–36.
5.
Wu AM, Till JE, Siminovitch L, McCulloch EA. Cytological evidence for a relationship between normal hemotopoietic colony-forming cells and cells of the lymphoid system. J Exp Med. 1968;127(3):455–64.PubMedPubMedCentral
6.
Spooncer E, Lord BI, Dexter TM. Defective ability to self-renew in vitro of highly purified primitive haematopoietic cells. Nature. 1985;316(6023):62–4.PubMed
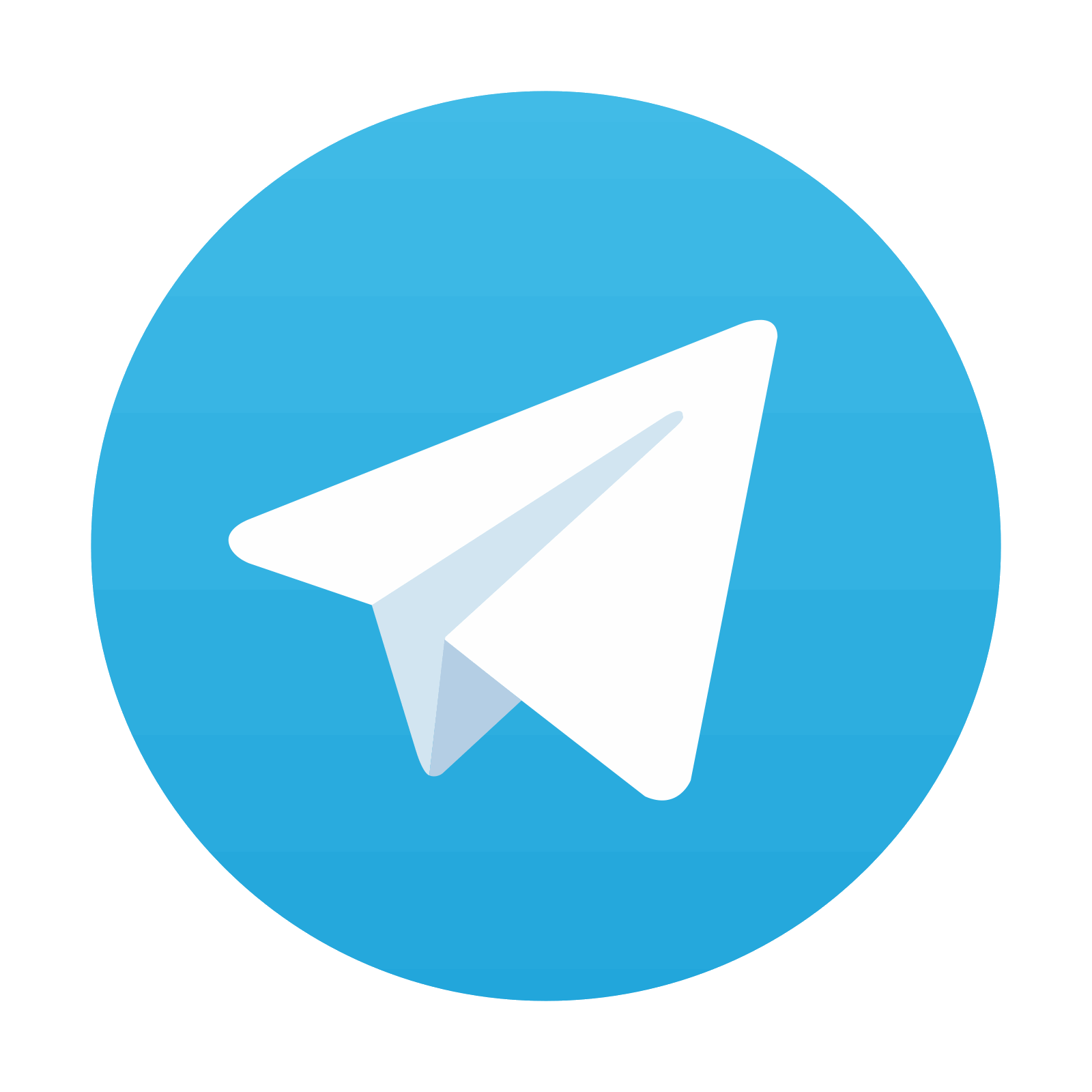
Stay updated, free articles. Join our Telegram channel
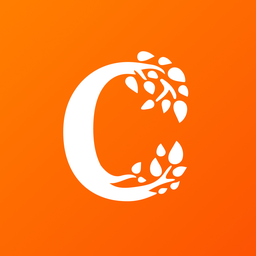
Full access? Get Clinical Tree
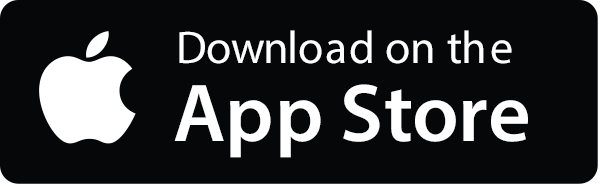
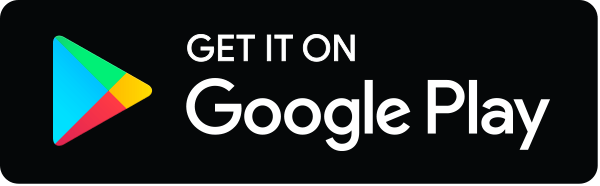