Chapter 1
Physiological Response to Trauma
Written by H. van Aswegen
Trauma is sometimes referred to as the hidden epidemic, as it tends to get much less media attention than the human immunodeficiency virus/acquired immunodeficiency syndrome (HIV/AIDS) pandemic but has an equally high mortality rate (Thomson, 2003). Trauma kills young people in the prime of their economically productive lives, and those that survive severe trauma often suffer from long-term health disabilities that impact their quality of life.
This chapter provides the physiotherapist with information about:
•The human immune system and its response to injury and inflammation.
•The impact of inflammation and sepsis on muscle function and structure.
•The response of the body to shock, different types of shock and the management of shock.
•The effect of shock and critical illness on blood oxygen content.
•The effect of trauma on blood glucose levels.
•The classification of patients with traumatic injuries to determine severity of illness, risk for mortality and morbidity estimation.
1.1. Human Immunity and its Response to Injury and Inflammation
Bodily injury sustained through traumatic events invariably leads to bleeding. Injury to blood vessel walls leads to haemostasis and coagulation to reduce the amount of blood lost from the circulation, followed by fibrinolysis in order to restore blood flow. Both of these processes are discussed in Sections 1.1.1. and 1.1.2. The coagulation system and immune system overlap, as bacteria that invade the body can be trapped inside blood clots that form during haemostasis. Immunity is the ability of the human body to protect itself against infiltration from infectious matter and foreign cells. The immune system is a complex system made up of many different immune system cells that continuously patrol the body to detect invasion of its barriers. An in-depth discussion of human immunity is beyond the scope of this book and the reader is referred to other texts for such detailed information. The immune system consists of non-specific as well as specific defences, which are discussed in Sections 1.1.3. and 1.1.4.
1.1.1. Haemostasis
The normal physiological response to blood loss as a result of injury to the vascular wall is a process called haemostasis. Blood changes from a liquid to a solid state during haemostasis. Haemostasis is made up of a sequence of events that are initiated within 20 seconds of vascular wall injury. The first step in this sequence is blood vessel constriction to reduce blood flow. This is followed by adherence of circulating platelets to the vessel wall at the site of injury. Platelets are activated by the endothelial cell injury and form a cluster to initiate the formation of a clot. A series of enzyme reactions occur, which involve coagulation proteins produced through intrinsic and extrinsic coagulation pathways to produce the enzyme thrombin. Thrombin is the key enzyme for coagulation. Fibrin is subsequently produced to form a stable haemostatic clot. The main function of haemostasis is therefore to maintain the integrity of the circulatory system. The haemostatic process can, however, be disrupted by inflammation and infection, resulting in uncontrolled bleeding and may lead to significant morbidity and even mortality (see septic shock in Section 1.2.3.3.3.) (Chan and Paredes, 2013).
1.1.2. Fibrinolysis
Fibrinolysis is the process of the restoration of blood flow by changing blood from a solid to a liquid state. As the integrity of the blood vessel wall is restored, endothelial cells secrete tissue plasminogen activators to start dissolving the clot. Plasminogen breaks up the fibrin in the clot and dissolves the clot, and during this process blood flow is restored. The fibrinolytic system can also be disrupted by inflammation and infection, resulting in uncontrolled thrombus formation and may lead to significant morbidity and mortality (see septic shock in section 1.2.3.3.3.) (Chan and Paredes, 2013).
1.1.3. Non-specific defence systems
Non-specific defences of the body include barriers such as the skin and mucous membranes, inflammatory reactions characterised by the stimulation of phagocytes, mast cells, eosinophils and natural killer cells. Protective proteins (such as interferon) also form part of the non-specific defences of the body and are activated when microbes enter the body. Protective proteins attract phagocytes to the scene of the infection in order to devour these microbes (Kapasi, 2006; Van Dyk, 2008) (Fig. 1.1).
1.1.4. Specific defence systems
The specific defences of the body consist primarily of white cells, of which the T-lymphocyte and B-lymphocyte activities are most important. The T-lymphocytes represent the cellular immune system and B-lymphocytes represent the humoral immune system. T-lymphocytes are responsbile for the destruction of foreign cells and coordinate the overall immune response of the body. Stimulation of the T-lymphocytes leads to the release of CD4 cells, which migrate via the blood stream to the site of infection and destroy the foreign invaders. CD4 cells also stimulate other immune system cells to mount the attack against the foreign cells. B-lymphocytes are responsible for the production of antibodies. These anitbodies, immunoglobulins, are deposited onto antigens (foreign substances that invade the defence barriers of the body), neutralise their actions and mark them for destruction by other immune system cells (Kapasi, 2006; Van Dyk, 2008) (Fig. 1.1).
1.1.5. Activation of the body’s defence systems
Local and systemic responses to trauma are activated by various factors, such as injury, surgery, dehydration, sepsis and acute medical illness. Traditionally it was thought that acute injury led to the loss of lean body and skeletal mass and preceded the process of recovery and wound repair. Recently the traditional view of the systemic response to injury has been expanded. Inflammation, as a result of injury, plays an important role in changing muscle structure and function during critical illness and is expanded on below. Inflammation consists of humoral and cellular responses, as mentioned above, and is driven by cytokine activity.
1.1.5.1. Cytokines
Cytokines are cell-signalling protein molecules that are secreted by a large number of cells of the immune system. Cytokines are produced by T-lymphocytes, macrophages, endothelial and epithelial cells. These cytokines include interleukin (IL) 1, IL-6 and tumour necrosis factor-alpha (TNF-α). Certain cytokines have a pro-inflammatory effect (IL-1, IL-6 and TNF-α) and others have an anti-inflammatory effect (IL-4, IL-10, IL-13 and IL-1 receptor antagonist). Cytokines have a systemic immunomodulating effect and, during infection or inflammation, proinflammatory cytokines signal T-cells and macrophages to travel to the site of infection and, in turn, as a result of the cell stimulation, the T-cells and macrophages produce more pro-inflammatory cytokines. Under healthy conditions this feedback loop is kept in check by the body through the release of anti-inflammatory cytokines that have an immunosuppression effect (Fig. 1.2); however, this balance between pro-inflammatory and anti-inflammatory activity is lost as a result of severe injury (Mukhopadhyay et al., 2006; Schroeder et al., 2009).
1.1.5.2. Systemic inflammatory response syndrome and sepsis
Worsening (exacerbation) of the inflammatory reaction in patients with trauma-related injuries can lead to the development of systemic inflammatory response syndrome (SIRS) due to an imbalance in the production of pro-inflammatory cytokines. Anti-inflammatory cytokines are released to restore this imbalance, but over-activation leads to either a compensatory anti-inflammatory response or a mixed antagonist response, which leads to post-traumatic immunosuppression (Hietbrink et al., 2006; Osuchowski et al., 2006; Schroeder et al., 2009). The patient’s immune system is in disarray and the body becomes very susceptible to infection, which might result in septic syndrome and ultimately multiple organ dysfunction syndrome (MODS) (Hietbrink et al., 2006; Osuchowski et al., 2006; Schroeder et al., 2009) (Fig. 1.3).
The American College of Chest Physicians and Society of Critical Care Medicine define SIRS as a systemic inflammatory response to various severe clinical insults such as burns, haemorrhage, ischaemia, inflammation and trauma. It is not always related to infection. Sepsis, on the other hand, is defined as a systemic response to infection (Clarke, 2003). Sepsis and SIRS are diagnosed according to the criteria outlined in Table 1.1; although the criteria are similar for both, diagnosis is made under different clinical circumstances, as mentioned above.
Table 1.1:Criteria for diagnosis of SIRS and sepsis.
•Temperature >38°C or <36°C •Heart rate >90 beats/minute •Respiratory rate >20 breaths/minute or partial pressure of carbon dioxide in arterial blood (PaCO2) <32 mmHg •White blood cell count >12,000 cells/mm3 or <4,000 cells/mm3 |
SIRS is diagnosed in the presence of two or more of the above after severe clinical insults to the body. Sepsis is diagnosed in the presence of two or more of the above in the presence of infection.
The presence of sepsis together with dysfunction of one or more organs is defined as severe sepsis. Organ dysfunctions can include: acute lung injury; blood clotting abnormalities; thrombocytopenia; altered mental status; failure of the kidneys, liver or heart; or the presence of lactic acidosis with hypoperfusion. Severe sepsis is caused by bacteraemia in 50% of cases, but in up to 30% of cases no microbial cause may be found for the sepsis. Severe sepsis in the presence of cardiovascular failure is defined as septic shock (Nguyen et al., 2006) (refer to Section 1.2.3.3.3. for a detailed description of septic shock).
1.1.6. Musculoskeletal changes associated with inflammation
It is normal for the body to have a surge in circulating cytokine levels during its immune response to injury or infection. These elevated cytokine levels, however, trigger secondary organ reactions that promote tissue damage and dysfunction. Sepsis is directly related to the development of myopathy, which involves the peripheral as well as respiratory muscles.
1.1.6.1. Muscle protein breakdown and atrophy
Pro-inflammatory cytokines such as IL-1 and TNF-a contribute to the development of symptoms such as fever, malaise and loss of appetite and lead to ‘capillary leak’. These cytokines contribute to muscle protein breakdown, cell death and reduction of muscle mass in the critically ill patient. Interleukin-6 concentrations are higher in patients with greater tissue trauma (high injury severity scores) and higher IL-6 concentrations are associated with adverse patient outcomes. Interleukin-6 levels also tend to be higher in patients with septic shock than in those with other types of shock. Interleukin-6 has been shown to stimulate the production of IL-1 and TNF-α until the patient’s condition becomes chronic, and then changes activity by slowing down the production of these cytokines; however, persistently high levels of IL-6 in the blood circulation have been associated with increased mortality in patients who are critically ill due to trauma-related injuries. Low levels of anti-inflammatory cytokines such as IL-10 in critically ill patients has been associated with excessive inflammation and muscle damage (Winkelman, 2004; Winkelman et al., 2007; Callahan and Supinski, 2009; Jawa et al., 2011).
1.1.6.2. Muscle weakness
Reactive oxygen species (ROS) are chemically reactive molecules that contain oxygen. They are produced as a natural byproduct to the metabolism of oxygen in the body. Small amounts of ROS form part of the internal defence system of the healthy human body. During illness, elevated levels of ROS that circulate through the body, as a result of cytokine stimulation, have been linked with decreased myofilament function at the muscular level, leading to muscle weakness (Fig. 1.4). Tumour necrosis factor-α has been linked with similar effects on muscle function, due to induced contractile dysfunction at the myofilament level. Reactive oxygen species mediate mitochondrial dysfunction in the skeletal muscle of patients with sepsis, which leads to muscle weakness (Winkelman, 2004; Callahan and Supinski, 2009).
The imbalances between pro-inflammatory and anti-inflammatory cytokine activity and elevated levels of circulating ROS and their effects on the musculoskeletal system offer an explanation for the commonly observed atrophic appearance of critically ill patients in addition to the effects of immobility on muscle function. Winkelman et al. (2007) suggested that low levels of physical activity in critically ill patients (rolling in bed, passive range of motion exercises) may assist with restoration of the imbalance between pro-inflammatory and anti-inflammatory cytokines. This suggestion is, however, based on a small sample size, and confirmation of findings are needed through large clinical trials.
The information shared in this section underscores the importance of the implementation of an appropriate early rehabilitation plan for a patient who suffers traumatic injury and critical illness. Such a plan should aim to address issues related to muscle weakness and joint range of motion as soon as the patient wakes up from sedation and is able to cooperate and participate in rehabilitation.
1.2. Shock and its Effects on the Human Body
1.2.1. What is shock?
The cardiovascular system is made up of the heart, the vascular network consisting of arteries, capillaries and veins, and blood. The heart propels the blood (with blood pressure as the driving force) and the vascular network distributes and collects it. These various components of the cardiovascular system are dependent on each other, and if one area malfunctions, the others compensate to return blood pressure to normal. One of the first signs of the development of shock is the presence of hypotension. Hypotension is a sign of cardiovascular insufficiency and indicates that either the heart is not working properly, there is not enough blood in the cardiovascular system or there is a malfunction of the distribution of blood through the body. Hypotension has caused shock when a patient presents with confusion, oliguria and/or lactic acidosis (signs of end organ insufficiency). Patients who have suffered trauma often develop shock due to severe blood loss, myocardial contusion, cardiac tamponade, tension pneumothorax or spinal injury. Shock is a circulatory failure that leads to inadequate tissue perfusion and end organ injury due to lack of oxygen supply at the cellular level. Generalised cellular damage occurs as a result. In the early stages of shock, cells extract more oxygen from each unit of blood and are able to maintain their oxygenation as oxygen delivery starts to decrease. This compensatory mechanism, however, becomes insufficient when oxygen delivery falls below a critical level.
1.2.2. Organ responses to blood loss
The ability of organs to withstand blood loss varies according to the amount of blood lost and the success of resuscitation and surgical interventions. The prognosis of the patient depends on their severity of injury, age and pre-existing illnesses (Schroeder et al., 2009).
1.2.2.1. Cardiovascular system
The heart rate and blood pressure responses to blood loss are directly related to the amount of total blood volume loss that occurs. Small children are more dependent on heart rate than blood pressure maintenance to compensate for blood loss. A loss of up to 15% of total blood volume in an adult has minimal physiological effects on the cardiovascular system. A loss of 15–30% total blood volume results in an elevated heart rate as well as an elevation in diastolic blood pressure (Garrioch, 2004). The rise in diastolic blood pressure occurs as a result of vasoconstriction, induced by the sympathetic nervous system. Blood loss of 30–40% total volume results in tachycardia and a reduction in systolic and diastolic blood pressure. If more than 40% total blood volume is lost, the patient may present with bradycardia and, in extreme situations, unrecordable blood pressure. Such a situation would be life-threatening (Garrioch, 2004).
In relation to the myocardium, myocardial contractibility may be influenced by traumatic injury, tamponade, mitochondrial calcium losses or nitric oxide production, and this might contribute to a reduction in cardiac output as well as stroke volume. Inappropriate vasodilatation as a result of sepsis or spinal shock might give rise to a reduction in peripheral vascular resistance, leading to a hypotensive state (McLuckie, 2003).
Inadequate restoration of circulating blood volume (low-perfusion state) is associated with the development of inflammation and the release of inflammatory cytokines throughout the circulatory system. The increased cytokine activity causes membrane injury to many vessels in the body, leading to leakage of plasma into the interstitium, which results in a loss of circulating volume and development of generalised oedema (Garrioch, 2004).
1.2.2.2. Central nervous system
A loss of more than 30% of total blood volume is accompanied by mental confusion and anxiety and, in severe cases, unresponsiveness (Garrioch, 2004).
1.2.2.3. Pulmonary system
In relation to the pulmonary system, hyperventilation occurs early during shock and is related to the development of lactic acidosis. Respiratory rate may increase to more than 20 breaths per minute as a result of lack of tissue oxygenation when 15–30% of total blood volume is lost. Persistently high respiratory rates would accompany blood loss of more than 30% total blood volume (Garrioch, 2004).
Persistent low-perfusion states, which may result from inadequate restoration of circulating blood volume, will lead to the development of inflammation, as discussed above. Systemic inflammatory response syndrome results in capillary leak associated with the development of acute respiratory distress syndrome (ARDS) manifested by progressive posterior lung segment atelectasis (Schroeder et al., 2009). Acute respiratory distress syndrome is defined as a syndrome of acute, diffuse lung inflammation and increased alveolar-capillary membrane permeability associated with clinical signs of decreased oxygenation, decreased lung compliance and increased physiological dead space. Characteristic radiological changes are bilateral radiographic opacities. Oxygenation is impaired in the absence of cardiac dysfunction (Costa and Amato, 2013). Respiratory muscle fatigue from muscle hypoperfusion and increased energy expenditure from breathing with heavy fluid-filled lungs may lead to respiratory failure.
1.2.2.4. Peripheries
In adults who have lost 15–30% total blood volume, a degree of peripheral shut down may be observed, which is characterised by cool extremities and peripheral cyanosis. Blood volume loss of more than 30% leads to the development of poor peripheral perfusion, and the patient will present with a pale appearance (Garrioch, 2004).
1.2.2.5. Renal system
With regard to the renal system, oliguria occurs due to renal ischaemia when autoregulation fails, or due to antidiuretic hormone and aldosterone secretion, which might result in fluid retention. Blood loss of more than 30% total volume results in negligible urine output (Garrioch, 2004).
1.2.2.6. Skeletal and splanchnic systems
In the early stages of shock the skeletal muscles and splanchnic organs (visceral organs such as gastrointestinal tract, liver, pancreas and spleen) are adversely affected by oxygen deprivation. The shock state delays mitochondrial activity and blocks the pathways of cellular energy production. This results in the development of lactic acidosis, which is a sign of widespread inadequate tissue perfusion. Splanchnic ischaemia occurs as vital organs such as the brain, heart, lungs and liver are preferentially perfused (Garrioch, 2004).
1.2.3. Types of shock
A person who has suffered trauma-related injuries is at risk of the development of shock. Various types of shock can be identified.
1.2.3.1. Cardiogenic shock
A person who has suffered trauma may be at risk for the development of cardiogenic shock when left ventricular function is impaired by injury, such as contusion of the ventricle during a motor vehicle accident or as a result of a fall from a height. Impaired left ventricular function leads to decreased stroke volume, decreased cardiac output, low blood pressure and inadequate tissue perfusion. The sympathetic nervous system response to low blood pressure is vasoconstriction, so increased systemic vascular resistance develops. Clinical presentation of the patient includes cool peripheries, decreased urine output and sweating (Parrillo and Dellinger, 2008).
1.2.3.2. Hypovolaemic shock
Hypovolaemic shock may develop in a person who has suffered trauma due to blood loss from organ and tissue injury or loss of blood plasma from burns. The decreased intravascular volume leads to decreased venous return, decreased stroke volume, decreased cardiac output, decreased blood pressure and results in inadequate tissue perfusion. Systemic vascular resistance is increased due to the sympathetic nervous system response to low blood pressure. The clinical presentation of the patient is similar to that described in Section 1.2.3.1. for cardiogenic shock (Parrillo and Dellinger, 2008).
1.2.3.3. Distributive shock
Distributive shock occurs when peripheral vascular dilatation causes a drop in systemic vascular resistance. The most common causes of distributive shock are neurogenic shock, anaphylactic shock and septic shock. A description of each of these types of shock is provided below. The clinical presentation of a patient with distributive shock includes warm peripheries, bouncing pulses, altered mental status, oliguria and lactic acidosis (Parrillo and Dellinger, 2008).
1.2.3.3.1. Neurogenic shock
A person who has suffered acute spinal cord injury or traumatic brain injury is at risk for the development of neurogenic shock. Neurogenic shock is the loss of sympathetic control of blood vessels, which results in massive dilatation of arterioles and venules throughout the body. Arteriolar dilation leads to reduced peripheral vascular resistance, resulting in arterial blood pooling which contributes to the decreased amount of blood returning to the heart. Venous dilation leads to venous blood pooling, which results in decreased stroke volume, decreased cardiac output and blood pressure and inadequate tissue perfusion.
1.2.3.3.2. Anaphylactic shock
A person who has suffered trauma may be at risk for the development of anaphylactic shock if he/she has an allergic reaction to blood products or drugs given during resuscitation in the emergency department, theatre or intensive care unit (ICU). Anaphylactic shock is caused by severe allergic antigen–antibody reactions. The patient presents with massive interstitial oedema due to increased capillary permeability as well as hypovolaemia due to venous and arteriolar vasodilation.
1.2.3.3.3. Septic shock
Sepsis is a systemic response to gram negative or gram positive bacterial or fungal infection, as discussed previously. Septic shock is defined as severe sepsis in the presence of persistent hypotension unexplained by other causes (cardiovascular failure). The patient’s immune system attempts to fight this infection by activating monocytes, macrophages and neutrophils. These cells interact with the vascular endothelial cells. The cellular activation and endothelial disruption leads to the release of multiple chemicals into the blood stream, including cytokines, ROS, nitric oxide, platelet-activating factor and vasodilators. Certain toxins such as endotoxins and exotoxins are produced, which contribute to the damage of organs and tissues. The toxins and chemicals cause microvascular injury, vasodilation of the capillary bed, capillary leak and interstitial oedema, thrombosis, tissue ischaemia and decreased circulating blood volume. Microvascular injury leads to decreased oxygen delivery and consumption at both cellular and tissue levels. Tissue ischaemia leads to various organ dysfunctions, as mentioned in Section 1.2.2. Thrombocytopenia (diminished platelet count) often leads to the development of disseminated intravascular coagulation. This signifies the disarray of the coagulation and fibrinolytic systems, which lead to spontaneous bleeding from organs, tissues, cavities and any venupuncture sites, as well as microvascular occlusion from excessive fibrin formation. Disseminated intravascular coagulation is associated with MODS and poor patient outcome (Nguyen et al., 2006; Nguyen and Smith, 2007) (Fig. 1.3).
1.2.3.4. Obstructive shock
This type of shock is caused by obstruction to cardiac filling. One of the most common causes of obstructive shock is cardiac tamponade, such as occurs with wounds to the heart from penetrating injury. The collection of blood in the pericardium prevents the ventricles from expanding fully. Obstructive shock may also develop due to tension pneumothorax or massive pulmonary embolus (Parrillo and Dellinger, 2008).
1.2.3.5. Refractory shock
Refractory shock is also referred to as irreversible shock. At this stage organs have failed and shock cannot be reversed, despite the administration of seemingly adequate therapy. Cardiac function is impaired by inadequate myocardial blood supply and by toxins released by inadequately perfused organs and tissues. Brain damage and cell death ensues (Parrillo and Dellinger, 2008).
1.2.4. Management of shock
The initial management of a patient with shock consists of the restoration of oxygen delivery to tissues in the form of oxygen therapy and, if required, mechanical ventilation with low tidal volumes. Restoration of blood volume involves the intravenous administration of crystalloid or colloid fluids and transfusion of red blood cells if indicated. The administration of vasoactive medication assists with the restoration of blood pressure and inotropic drugs assist with the restoration of stroke volume. Early goal-directed therapy is recommended in the emergency department management of a patient with septic shock. This is a management algorithm that is usually instituted and completed within the first six hours of the patient’s presentation in the department. Early goal-directed therapy is shown to improve patient outcome significantly. Early detection of the site of infection and implementation of source control measures assists with the further improvement of patient outcome. Source control includes: drainage of an abcess or effusion, wound debridement and initiation of antimicrobial therapy. Patients with refractory shock may be given low-dose corticosteroid therapy in addition to the therapy mentioned above in an attempt to reverse the shock state. The addition of recombinant human-activated protein C therapy for patients with septic shock who present with an APACHE II >25 (see Section 1.6.1.1. for a discussion on APACHE score) has improved survival rates significantly. Recombinant human-activated protein C is used to restore balance to the abnormal coagulation and fibrinolytic pathways associated with septic shock (Nguyen et al., 2006; Nguyen and Smith, 2007).
1.3. Factors that Influence Blood Oxygen Content
Shock, as well as critical illness, can affect the oxygen content of blood due to loss of blood and anaemia. The oxygen content of blood is determined by the amount of oxygen dissolved in the blood plasma and the amount of oxygen bound to haemoglobin. In healthy individuals, low levels of haemoglobin are quickly corrected by an increase in the production of erythropoietin, a glycoprotein hormone that regulates the production of red blood cells (erythropoiesis). Dietary iron is bound to a protein called transferrin and circulates through the body. Excess iron is stored intracellularly through binding with another protein called ferritin. Scharte and Fink (2003) studied red blood cell physiology in critical illness in depth and have stated that a decrease in erythropoietin production response has been observed in patients who suffered trauma. They also stated that low levels of serum iron and transferrin and high levels of ferritin are associated with critical illness, which means that less free iron is available for the production of red blood cells. This reduction in serum iron levels has been linked to the activity of proinflammatory cytokines such as IL-1, IL-6 and TNF-α. These cytokines directly provoke the production of ferritin and thereby enhance iron storage intracellularly, which further limits the availability of iron for red blood cell production. Oxygenation is also influenced by phlebotomy (line insertion, sampling for arterial blood gas analysis) in the ICU, which accounts for up to 20% of total blood loss (Scharte and Fink, 2003). This illustrates that a person who has suffered trauma and become critically ill as a result might present with hypoxaemia not only related to chest injuries sustained but also due to a reduction in total blood volume resulting from the processes described around critical illness and ICU procedures.
1.4. Effect of Trauma on Blood Glucose Levels
The initial response of the human body to trauma is to access energy from internal (endogenous) fat oxidation. Fat provides most of the body’s energy requirements during the period in which no food is ingested. Fat is mobilised from fat stores and converted to free fatty acids and glycerol. These are then circulated to tissues such as the large muscles, which can burn the fatty acids directly. Blood sugar, however, starts to rise slowly and insulin production from the pancreas is inhibited. It has been reported that hyperglycaemia may contribute to a patient’s mortality rate (Schroeder et al., 2009). Poor glycaemic control is associated with increased morbidity and mortality in critically ill trauma patients and is more prevelant in non-diabetic patients (Gale et al., 2007). A greater occurrence of urinary tract infections and complications in non-diabetic trauma patients with poor glycaemic control has also been found (Eakins, 2009). Hyperglycaemia plays a role in the activation of blood coagulation pathways and may put the patient at risk of developing acute thrombosis. Tight glucose control through the administration of insulin therapy has been shown to have a beneficial effect on short-term morbidity and mortality in critically ill patients (Pittas et al., 2004) and today should form part of the standard ICU care of adult patients who have suffered trauma.
In light of this information, an important role of the physiotherapist in the ICU is daily screening of the patient for signs and symptoms of the development of deep venous thrombosis and bringing this to the attention of the interprofessional ICU team.
1.5. Clinical Case Scenario
This scenario incorporates information from all the sections discussed above to demonstrate acute care management of a patient with traumatic injury.
A 33-year-old woman made use of public transport as she travelled home from work. On the way home, the minibus taxi that she was travelling in burst a rear tyre and collided with an oncoming bus. The woman sustained a pelvic fracture and blunt injuries to her abdominal region. She was transported by ambulance to the nearest trauma centre.
In the casualty department she presented with hypovolaemic shock due to internal blood loss from the pelvic fracture and blunt abdominal trauma. Oxygen therapy was started using a partial re-breathing mask as she was still awake and alert. Early goal-directed therapy was initiated and she was taken to theatre for surgical intervention. She was intubated in theatre and central venous and arterial lines were inserted. The pelvic fracture was surgically managed with an external fixator and a laparotomy incision was made to gain access to organs in the abdominal cavity and control the internal bleeding. Afterwards she was transferred to the ICU for monitoring and further management.
In the ICU she remained intubated and was mechanically ventilated with low tidal volumes in order to prevent ventilator-associated lung injury. She presented with low blood pressure due to blood loss from her injuries and surgical interventions in theatre. Intravenous fluids were administered together with vasoactive drugs to restore her blood pressure to acceptable levels. Analgesic and sedative medications were administered, as well as insulin therapy to maintain glucose control. During her stay in the ICU, arterial blood samples were taken every four hours for blood gas analysis and on several occasions blood samples were taken for full blood count, urea and electrolyte analysis and microbiological cultures. As a result of her injuries and the resultant surgery she presented with SIRS, which escalated to sepsis due to wound infection six days after admission to the ICU. This was managed with the administration of antimicrobial therapy, to which she had a favourable response.
Physiotherapy intervention in the ICU while this patient was sedated would consist of prevention or management of respiratory complications, optimisation of oxygenation, passive maintenance of muscle length and joint end of range motion (within precautions and contraindications posed by the pelvic fracture; see Chapter 7) and regular screening of the patient for the development of deep venous thrombosis. As she regains consciousness, the focus of physiotherapy management would shift to active patient participation in rehabilitation in order to address issues related to muscle atrophy and weakness as a result of critical illness and prolonged immobilisation. Active weightbearing through the pelvis with mobilisation would be considered after discussion with the orthopaedic team. The patient would be monitored closely for signs of shortness of breath and unexpected changes in vital signs during chest physiotherapy, exercise rehabilitation and mobilisation.
1.6. Classification of Patients with Traumatic Injuries
The classification of patients with severe injuries on admission to hospital is important. The determination of their severity of illness and risk for mortality guides medical and surgical decision-making in their care pathways. The classification of patients is also important for data capturing in ongoing research studies at trauma centres worldwide in order to objectively compare patient populations and treatment outcomes. Physiotherapists may encounter these classification scoring systems during their daily work in an ICU setting or when reading critical care and trauma literature, and therefore an explanation of the commonly-used scores in the trauma care environment is provided below.
1.6.1. Severity of illness scoring systems
The risk of mortality after admission to the ICU is influenced by increasing age, severity of acute illness, pre-existing medical conditions such as cancer, renal failure or immunosuppression, and emergency admission to the ICU. The severity of illness scoring systems were developed in the 1980s to help predict the outcome of patients with critical illness.
1.6.1.1. APACHE II and SAPS III
The Acute Physiology and Chronic Health Evaluation (APACHE) score was developed in 1981 to describe accurately the severity of illness of various groups of patients. This was done utilising 34 individual variables (Wong and Knaus, 1991). The APACHE II was developed in 1985 and represented a simplified version of the APACHE score. APACHE II is the most widely used and studied scoring system to date. It is composed of three parts, namely: a) the acute physiology score, composed of 12 laboratory and physical variables; b) the patient’s age; and c) a chronic health evaluation. The APACHE II is calculated within the first 24 hours of ICU admission and the maximum score is 71. The higher the score, the greater the risk for mortality (a score of 25 is associated with a predicted mortality of 50%). Unfortunately the APACHE II does not have a component for anatomical injury and therefore its ability to predict outcome in trauma patients in the ICU is questionable (Bouch and Thompson, 2008).
The Simplified Acute Physiology Score (SAPS) was developed in 1984 and consisted of the evaluation of 14 physiological variables. The SAPS was subsequently upgraded to SAPS II and SAPS III, both of which evaluate 12 physiological variables and include data related to pre-exisitng health status and age. Similar to the APACHE II, the SAPS III is calculated within the first 24 hours of ICU admission (Bouch and Thompson, 2008).
1.6.1.2. Anatomic and physiologic scores
Various anatomic scores, such as the abbreviated injury score (AIS) and the injury severity score (ISS), were developed to predict mortality in patients who suffered traumatic injury. The AIS was a complex scoring system and formed the basis for the development of the less complex ISS. Various physiologic scores were also developed, such as the revised trauma score (RTS) and the SIRS score (Table 1.2). These scores use physiologic parameters to assess patient outcome. A SIRS score equal to or greater than two has been shown to be predictive of ICU admission and greater risk of mortality than a score less than two (Malone et al., 2001). Some limitations to the effectiveness of the RTS have been documented, especially in relation to accurate assessment of the Glasgow coma scale (GCS) component in patients who are intubated and mechanically ventilated.
Table 1.2:Systemic inflammatory response syndrome score.
Each component is assigned 1 point. |
•Fever or hypothermia (temperature >38°C or <36°C) •Tachypnoea (respiratory rate >20 breaths/minute or PaCO2 <32 mmHg) •Tachycardia (heart rate >90 beats/minute) •Leukocytosis or leukopenia (white cell count >12,000/mm3 or <4000/mm3) |
SIRS score can range from 0–4.
Table 1.3:Trauma and injury severity score (TRISS).
Online TRISS calculator can be accessed at http://www.trauma.org/js/trisscalc.html
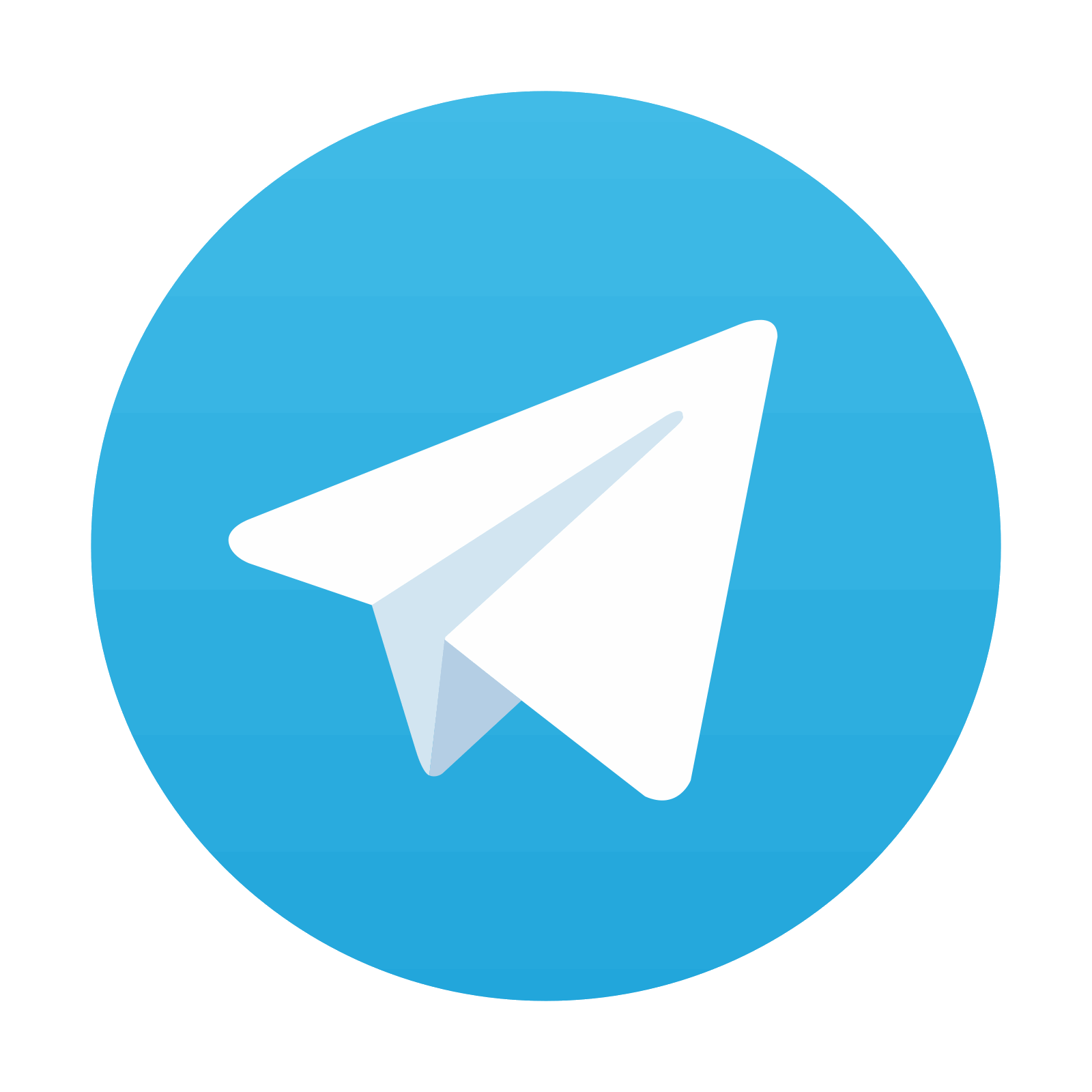
Stay updated, free articles. Join our Telegram channel
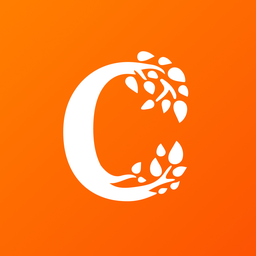
Full access? Get Clinical Tree
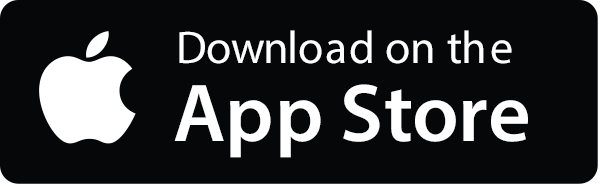
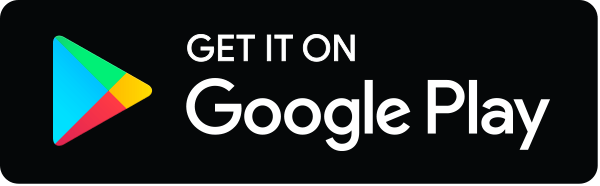