Figure 24–1.
Panel a: Representation of an HCN channel subunit, four of which together form a functional channel generating If current. Shown are the six membrane spanning segments, the pore loop and the cAMP binding domain (Modified from Robinson and Siegelbaum [9] with permission). Panel b: Spontaneous action potential recordings from single rabbit sinus node cells showing the effect of the β-adrenergic agonist isoproterenol (Iso, top) and the muscarinic agonist acetylcholine (ACh, bottom) on spontaneous rate and slope of phase 4 depolarization (Modified from Bucchi et al. [10]. With permission from Wolters Kluwer Health)
In addition to impulse initiation, the other key attribute of the sinus node is its autonomic responsiveness, which is dependent on modulation of intracellular cAMP levels by sympathetic and parasympathetic neurohumors. Critical to this modulation is the structure of the HCN channel, which has six transmembrane spanning domains, and incorporates a cyclic-AMP binding site near its carboxy-terminus [6, 9]. β-adrenergic catecholamines via a G-protein-transduced pathway operate through adenylyl cyclase to break down ATP to cyclic AMP, which then can interact with its HCN channel site to increase the rate of phase 4 depolarization and thus pacemaker rate (Fig. 24.1b) [10]. The increase in rate results from an increase in activation kinetics and a positive shift along the voltage axis of the current. In contrast, acetylcholine utilizes its G-protein-transduced pathway to brake the increase in rate by decreasing cAMP levels and reducing the rate of phase 4 depolarization. At higher concentrations, acetylcholine also increases the potassium current IK,Ach to accelerate repolarization, hyperpolarize the membrane potential and suppress phase 4 [11]. This further slows the rate of impulse initiation.
Although the information just reviewed highlights the importance of If to sinus node pacemaker function, the other components contributing to nodal impulse initiation are such that administering highly selective If –blocking drugs such as ivabradine only decreases heart rate by 10–30 % [12].
The other contributors of inward current during phase 4 include the Na/Ca exchanger and the inward Ca currents, ICa,L and ICa,T [6]. These contributors also are responsive to autonomic regulation via cAMP, in this case via PKA dependent phosphorylation either of these proteins or of other proteins involved in regulation of Ca2+ homeostasis that in turn modulate their function [13, 14]. Recent evidence suggests that there is an additional cAMP dependent interaction between If and these Ca2+ dependent currents and exchanger. This interaction arises from the fact that, unlike ventricular myocardium, sinus node expresses Ca2+-activated adenylyl cyclase isoforms [15, 16]. The presence of such isoforms provides a mechanism by which Ca2+ dynamics can influence – via cAMP levels – not only Na/Ca exchanger and Ca2+ currents, but also If [17].
The sinus node action potential itself is largely Ca-dependent (in contrast to the faster-rising Na-dependent action potentials of working myocardium). However, in some species and at some ages – particularly in settings where basal heart rate is high – fast inward Na current also is present [18, 19]. In these cases, the Na channel isoform is not the “cardiac” Nav1.5, but other isoforms (e.g. Nav1.1) that exhibit a less negative inactivation relation, thereby allowing the channels to operate at the membrane potential typical of the sinus node.
Finally, repolarizing current is provided via potassium channels, generating an outward current [6]. Hence, a balance between inward and outward currents determines sinus rate, and any intervention that increases inward or decreases outward current during diastole will increase pacemaker rate.
Biological Pacemakers
Three situations in which one might want to use biological materials as opposed to an electronic pacemaker are (1) repair or creation of a sinoatrial node in sick sinus syndrome with intact AV nodal function, (2) creation of a demand ventricular pacemaker in individuals with high degree or complete heart block and atrial fibrillation; (3) creation of an atrioventricular bridge in individuals with normal sinus node function and AV nodal disease. Note that the latter is not a biological pacemaker, but a biological alternative to electronic atrioventricular sequential pacing that could be combined with a biological pacemaker, if needed. This presentation will focus on biological pacemakers rather than on atrioventricular bridges.
In creating a biological pacemaker we recognize that any intervention that increases inward current or decreases outward current during diastole in an excitable cell might induce pacemaker function. We have characterized what we believe to be the optimal characteristics of a biological pacemaker as follows [3]: It should create a stable physiologic rhythm for the life of the patient, require no battery or electrode, and no replacement, compete effectively in direct comparison with electronic pacemakers, confer no risk of inflammation/infection or neoplasia, adapt to changes in physical activity and/or emotion with appropriate and rapid changes in heart rate, propagate through an optimal pathway of activation to maximize efficiency of contraction and cardiac output, and have limited and preferably no arrhythmic potential. We have deliberately set a high bar here because electronic pacemakers provide a standard for excellence as palliative therapy. Indeed, to exceed the success of electronic pacemakers, the biological pacemaker should represent cure, not palliation.
Biological pacing has incorporated two general approaches, that of gene therapy and that of cell therapy. Initial studies focused on gene therapy and generated transient successes, in keeping with the episomal delivery of the gene. While gene therapy remains an important field for investigation, cell therapies have also been explored. In this presentation we will discuss gene and cell therapies sequentially.
Gene Therapy
Initial Attempts at Biological Pacemaking
Initial attempts at biological pacing focused on sympathomimetic modulation of existing pacemaker current. Edelberg et al. [20] reasoned that since β-adrenergic catecholamines can increase sinus rate, overexpressing β-adrenergic receptors should enhance heart rate. Hence, they incorporated the gene encoding the β2-adrenergic receptor into a plasmid and injected this into porcine atrium in situ. Although the transfection was transient, they demonstrated both a faster atrial rate and an enhanced atrial rate response to catecholamine infusion in the injected animals. Concerns about this approach were: first, therapy would rely on the ability of the existing sinoatrial node (or other endogenous pacemaker) to respond to catecholamines. If the node were in any way damaged or diseased the response might be unpredictable. Second, catecholamines can be arrhythmogenic. However, as detailed below, a recent variant on this approach involves manipulating adenylyl cyclase to influence cAMP levels.
Miake et al. provided the first proof of concept experiments that alteration of ion channel function could create a biological pacemaker [21]. They reasoned that if repolarizing current were reduced, this would permit inward currents to depolarize the membrane during electrical diastole, resulting in a functioning biological pacemaker. To this end, they replaced three amino acid residues in the pore of the Kir2.1 gene that regulates the ion current, IK1 and created a dominant negative construct. This was presumed to form multimeric, nonfunctional channels with endogenously expressed wild-type Kir2.1 and/or Kir2.2. The construct and green fluorescent protein (GFP) were packaged in a replication-deficient adenoviral vector which was injected into the left ventricular cavity of guinea pigs. After 3–4 days IK1 was about 80 % reduced in isolated ventricular myocytes, and ventricular ectopic rhythms were seen on ECG. Recently this approach has been combined with HCN over-expression [22]. The major concern about reducing outward repolarizing currents was that it might excessively prolong repolarization, as was borne out in later experiments [23].
Using HCN to Create Biological Pacemakers
Proof of concept that increasing If in myocytes would lead to increases in pacemaker rate was provided by our group [24–26]. We elected to overexpress the HCN channel as the biological pacemaker because it not only carries the primary pacemaker current but because If activates on hyperpolarization and turns off at depolarized potentials. Therefore it has no effect to prolong action potential duration. Further, it directly binds cAMP [9], providing inherent autonomic responsiveness. After showing in rat ventricular myocytes in culture that If could be overexpressed by administering the HCN2 gene using a plasmid as the vector [24], we asked whether the channel expressed was autonomically regulated. Here, we infected ventricular myocytes with an adenoviral construct incorporating HCN2 and found cAMP regulation of the current was preserved, similar to that for wild-type If [25]. We also found that a β subunit, MiRP1, administered via a plasmid, increased pacemaker current density and kinetics of exogenously expressed HCN2 in xenopus oocytes [26] and in myocytes [27]. The final in vitro experiment involved HCN2 overexpression in neonatal myocytes in culture using an adenoviral vector. Spontaneous rate increased, suggesting a similar approach might result in biological pacemaking in situ [24, 25]. These results are demonstrated in Fig. 24.2.
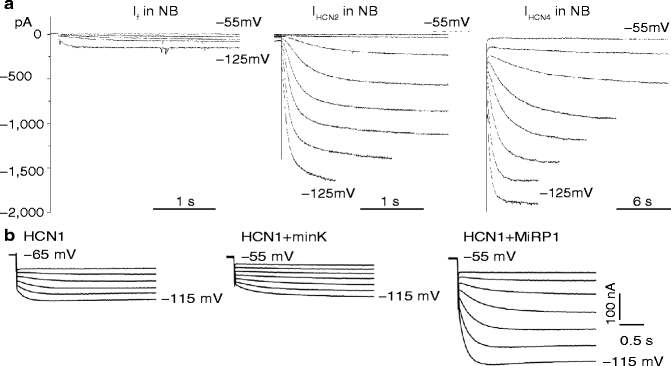
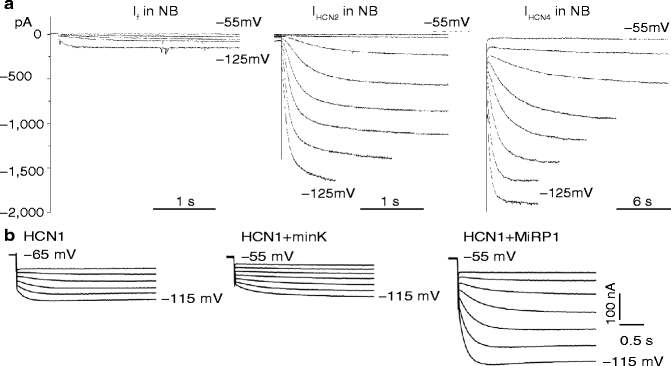
Figure 24–2.
Panel a: Representative tracings from newborn (NB) rat ventricular myocytes transfected with HCN genes. Left, native If in newborn myocyte; center, transfection with HCN2 gene; right, transfection with HCN4 gene. Note the markedly larger currents in center and right panels as compared to the left. Panel b: Effect of MiRP1 transfection to increase current in xenopus oocytes transfected with HCN2. Left represents HCN2, alone; center, HCN2 plus the β subunit minK; right HCN2 plus the β subunit MiRP1. Note the markedly larger current n the right panel (Modified from Qu et al. [24] and Yu et al. [26], with permission)
Our initial attempt at proof of concept in vivo involved injecting HCN2 into left atrial myocardium [28]. We used an adenoviral vector incorporating HCN2, with a second adenovirus expressing green fluorescent protein (to facilitate visualization of transfected cells). In terminal experiments we used vagal stimulation to induce sinoatrial slowing and/or AV nodal block, and studied the subsequent escape rhythms. The approach was successful and we pace-mapped the ectopic rhythms to the site of injection of the construct. We then isolated and studied cells from that region, which showed If at least 100-fold greater than native current [28].
Although this approach successfully demonstrated functional escape rhythms, we believed it important to test the concept in the ventricle. Hence, under fluoroscopic control, we injected the same construct into the left bundle branch system of intact dogs [29]. We again used vagal stimulation to slow sinus rate or induce atrioventricular block and found that HCN2-injected dogs developed escape rhythms whose rates were about 25 % faster than the idioventricular rates of control or sham injected (GFP without HCN2) animals (Fig. 24.3a, b) [29]. We then isolated left bundle branch fibers from the injection site and studied them with microelectrode techniques. They showed a significantly faster automatic rate than those generated at the same sites by tissues from control or sham-injected animals [29]. Current recordings from cells disaggregated from the same sites showed approximately 100-fold overexpression of If in HCN2 injected animals (Fig. 24.3c). Finally, immunohistochemistry demonstrated increased fluorescence with an anti-HCN2 antibody.
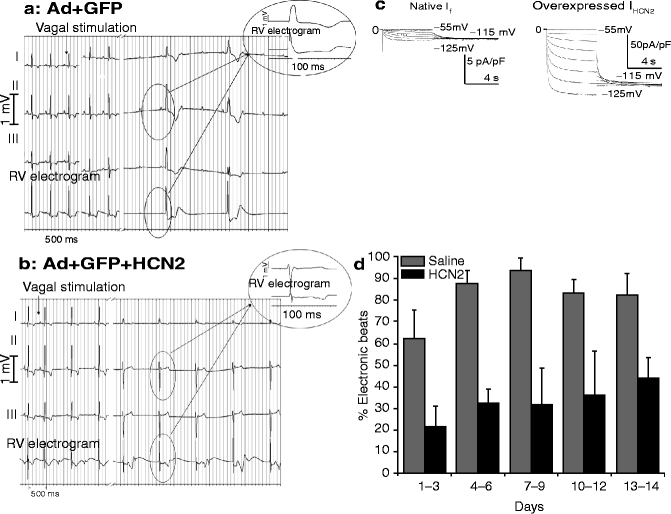
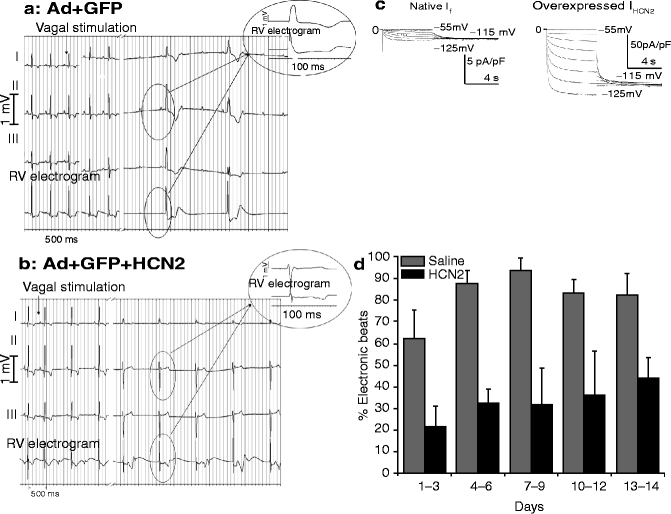
Figure 24–3.
Panels a and b are respectively Leads I, II and III and a right ventricular electrogram (top to bottom) of the ECGs of two different dogs. That in (a) was injected with an adenoviral vector carrying green fluorescent protein (GFP), alone; that in (b) received the same vector incorporating GFP plus the HCN2 gene. Animals were anesthetized 6 days after injection and the vagi isolated and stimulated (stimulation marked by the arrows). Interval between traces is 22 s in (a) and 5 s in (b). The animal that received GFP developed an idioventricular rhythm having a slower rate than that receiving GFP+HCN2. Insets show the temporal relationship between the lead II QRS complex and the electrogram. Panel c: If in a Purkinje myocyte isolated from the LBB of a control animal (left) and one receiving HCN2 (right). The current is markedly greater in the latter (note the vertical gains of 5 pA/pF on the left and 50 pA/pF on the right). Panel d: Percent of electronic beats as a function of days after injection into the LBB of saline or an HCN2 adenovirus (Modified from Plotnikov et al. [29] and Bucchi et al. [30], with permission)
In a subsequent study [30] we demonstrated that an HCN2 biological pacemaker implanted in the left bundle branch could function smoothly in tandem with an electronic pacemaker set at VVI 45 bpm. The biological pacemaker significantly reduced the incidence of electronic beats (Fig. 24.3d) while the electronic pacemaker’s memory function provided a record of pacemaker functionality. Finally, a recent study confirms that an HCN2 based biological pacemaker responds to natural physiological stimuli, in this case using arousal to induce an increase in rate [31].
Other experiments focused on fine-tuning pacemaker properties using mutant or chimeric constructs. For example, we have used the E324A mutant channel, incorporating a single point mutation at position 324 of the parent mHCN2 and found that this elicits a sequence of complex changes: first, there is less channel and current expression with the mutant than the parent HCN2; however there is a positive shift of both activation and cyclic AMP responsiveness: the result is expressed as a baseline rate not very different from that with HCN2 alone, but there is a greater rate response to catecholamines [30]. We also employed a chimeric HCN1/HCN2 channel which combined the transmembrane region of HCN1 with the cytoplasmic N- and C-termini of HCN2. The rationale was that the HCN1 component would provide faster gating kinetics while the HCN2 C-terminus, with that cAMP binding domain, would provide a robust cAMP response. This prediction proved correct, but the resultant biological pacemaker generated excessive rates, resulting in periods of ventricular tachycardia [32]. The HCN basis for the tachycardia was confirmed by the efficacy of ivabradine to terminate it.
Another group has employed an HCN1 mutation that deletes a portion of the S3–S4 linker, shifting activation positively [33]. The resultant pacemaker, implanted in atrial tissue, exhibited a good range of rates in a porcine model of SAN dysfunction and a demonstrable catecholamine response. This occurred despite the poor inherent catecholamine response of the HCN1 channel subunit, suggesting contributions from endogenous elements.
While interventions such as these provide means to optimize pacemaker constructs, no single gene construct based on HCN has yet achieved optimal results in terms of the basal or autonomically stimulated rate attained. This suggests that a combination gene approach may be necessary, and several initial attempts along that line have been pursued.
In considering combination gene approaches, Nature has given us at least two clues. The first derives from the observation that the sinus node expresses Ca2+-activated adenylyl cyclase (AC) isoforms [15, 16], which provides a possible intrinsic feedback mechanism between cytosolic Ca2+ changes and activation of transmembrane currents, in particular HCN based currents. We have pursued this by over-expressing AC1 (an isoform activated by Ca2+ in the submicromolar range [34]) in the canine left bundle branch in combination with HCN2. AC1+HCN2 expression resulted in a significantly greater escape rate and greater maximal rate following adrenergic agonists than HCN2 alone [35]. While we have not yet compared this to a pacemaker based on a different AC isoform, another group has studied the effect of AC6 over-expression (without HCN co-expression) [36]. This isoform, which is inhibited by Ca2+ in the micromolar range, was able to generate a biological pacemaker, but only in the setting of catecholamine stimulation.
A second idea for combination gene therapy derives from the observation that in animals with intrinsic high heart rate a Na channel, and in particular an isoform that is not inactivated at the typical membrane potential of the sinus node, contributes to diastolic depolarization [19, 37]. We explored this concept by overexpressing the Nav1.4 Na channel isoform along with HCN2 [38]. At low membrane potentials at which more than 50 % of cardiac Na channels are inactivated, cells expressing Nav1.4 generate action potentials having a high maximum rate of action potential upstroke [39, 40]. We administered combined SkM1/HCN2 to the left bundle branch of dogs in chronic heart block. Impulse initiation was improved over HCN2 or SkM1 alone, yielding basal rates of 70–100 bpm and maximal rates of 150–170 bpm [38]. While the mechanism underlying this combination approach requires further exploration, the result was a biological pacemaker operating in the normal physiological range and possessing a robust response to adrenergic stimuli.
Why Seek Alternatives to Gene Therapy?
An obvious advantage of the HCN-based approach to gene therapy is that it not only overexpresses members of the primary pacemaker gene family, but it does not induce excess prolongation of repolarization and it retains the function of autonomic responsiveness. However, there are problems with the viral vector approach: replication-deficient adenoviruses or naked plasmids represent episomal strategies and the construct administered is not incorporated into the cell’s genome. Such expression of biological pacemaking is transitory. Moreover, the viral vector induces inflammation and there is also the risk of infection. Finally, some viruses that might be expected to result in genomic incorporation of a pacemaker construct carry an already-demonstrated risk of neoplasia [3, 4]. Concerns such as these have led us and others to examine cell therapy approaches to biological pacemaking. These approaches have largely (albeit not exclusively) depended on xenografting human stem cells into animal hearts.
Cell Therapies
Human Embryonic Stem Cells
Research on embryonic stem cells has been proceeding for over 25 years, having been initiated by biologists interested in maturation during early stages of development [41, 42]. Research on human embryonic stem cells is far more recent. The major contribution to date in human embryonic stem cell research on biological pacemaking has been provided by the Gepstein group [43–45]. They have used embryonic stem cells to create a cardiogenic line that has been implanted into the hearts of immunosuppressed pigs in complete heart block. Their implantation has resulted in stable idioventricular rhythms that have persisted for months, and whose initiating current appears consistent with If.
Yet, questions remain regarding the long-term consistency of function as well as whether the current providing pacemaker function is If or a family of currents. A potential limitation of this approach is that the cells must be driven down a cardiac lineage that results in spontaneously active cardiac-like cells (as opposed to, e.g., ventricular like cells), and in fact the resulting cardiac-like population is quite heterogeneous [46]. Even with a pure population of pacemaking cells, the magnitude and kinetics of the diastolic current generated will be determined by the endogenous channels expressed in the cell. In contrast, expression of exogenous genes makes it possible to consider channel mutagenesis and/or combination therapy to optimize pacemaker function, as described above. This is one of the considerations that led us to explore the use of engineered adult mesenchymal stem cells, as discussed in the next section.
In addition, a major concern with embryonic stem cells is the need for immunosuppression: most physicians and patients faced with a decision to pace biologically and immunosuppress, versus to pace electronically and not immunosuppress would elect the latter approach. An alternative approach that does not require immunosuppression (but does not address the issue of optimization of pacemaker function) is creation of autologous biological pacemakers using induced pluripotent stem cells (IPSCs) [47, 48]. Retroviral administration of transcription factors to adult cells reprograms them, resulting in pluripotent IPSCs that can then be driven down a cardiac lineage in a manner analogous to ESCs. As with ESCs, concerns remain regarding possible tumorgenicity and control of the terminally differentiated state. Some of these concerns for IPSCs may be alleviated by more recent approaches, including ones that result in the direct reprogramming of fibroblasts to automatic, differentiated cardiomyocytes without involving a pluripotent intermediate or the use of viruses [48, 49]. However, concerns remain about abnormal phenotype in these cells, as reflected in changes at the chromosomal and subchromosomal levels [50, 51].
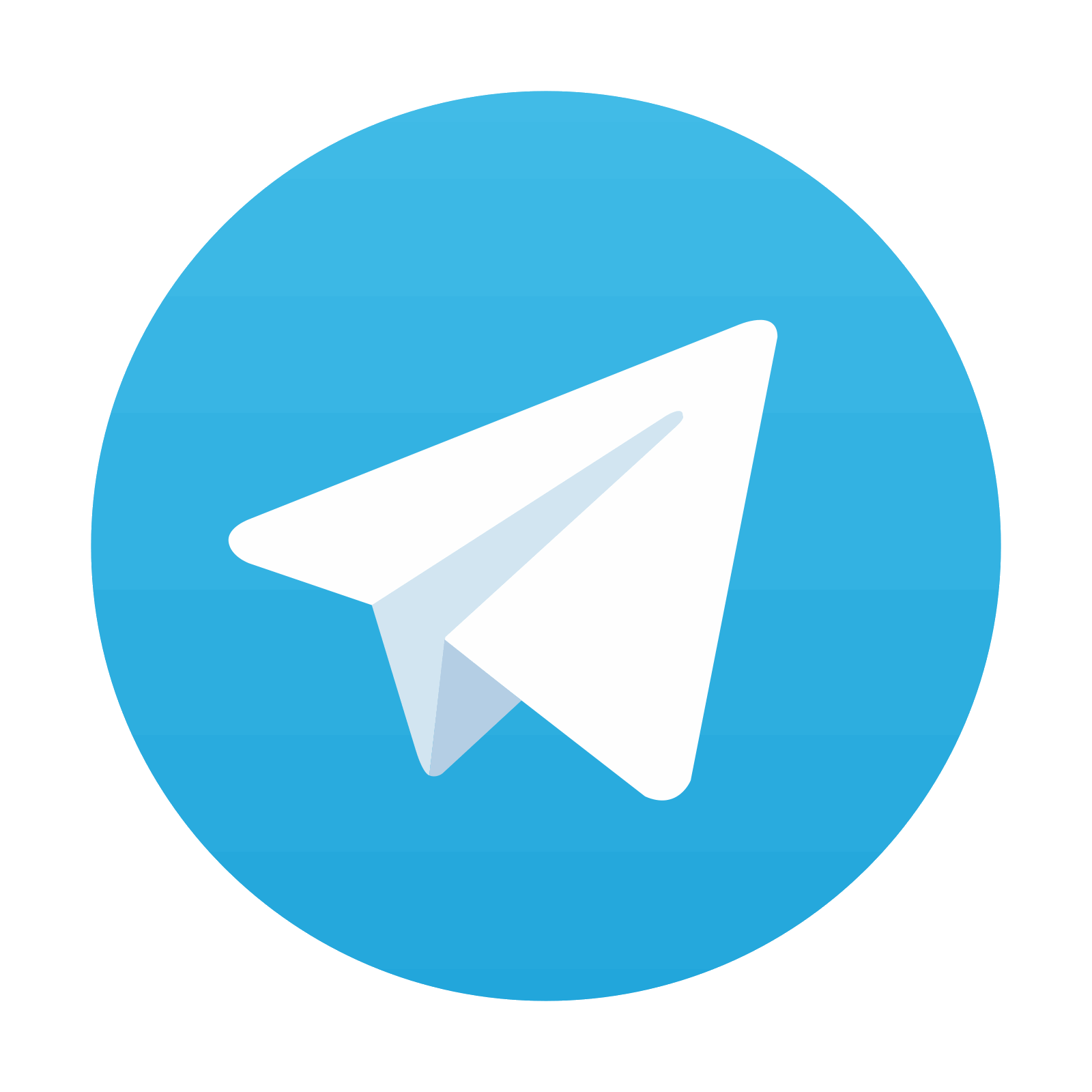
Stay updated, free articles. Join our Telegram channel
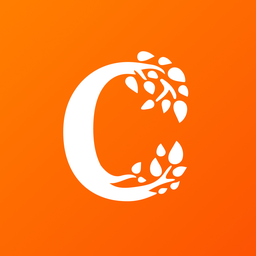
Full access? Get Clinical Tree
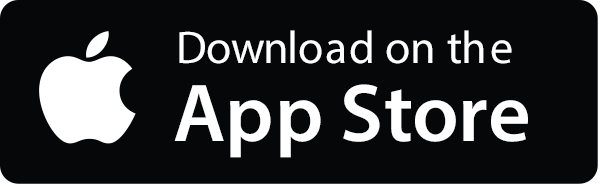
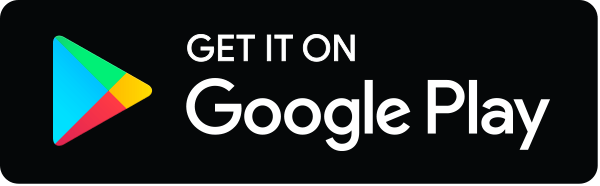