Fig. 1.1
Two- and three-dimensional transducers. Schematic drawing showing the main characteristics of two- (left panel) and three-dimensional (right panel) transducers
Modern 3DE matrix-array transducers are composed of about 3000 individually connected and simultaneously active (fully sampled) piezoelectric elements with operating frequencies ranging from 2 to 4 MHz and 5 to 7 MHz for transthoracic and transoesophageal transducers, respectively. To steer the ultrasound beam in 3D, a 3D array of piezoelectric elements needs to be used in the probe, therefore piezoelectric elements are arranged in rows and columns to form of a rectangular grid (matrix configuration) within the transducer (Fig. 1.1, right upper panel). The electronically controlled phasic firing of the elements in that matrix generates a scan line that propagates radially (y or axial direction) and can be steered both laterally (x or azimuthal direction) and in elevation (z direction) in order to acquire a volumetric pyramidal data set (Fig. 1.1, right lower panel). Matrix array probes can also provide real-time multiple simultaneous 2D views, at high frame rate, oriented in predefined or user-selected plane orientations by activacting multiple lines of piezoelectric elements within the matrix (Fig. 1.2).


Fig. 1.2
Multiplane acquisition using the matrix array transducer. From the left parasternal approach, the probe can be used to obtain simultaneous long- and short axis views of the left ventricle during the same cardiac cycle (right panels). From the apical approach, the probe can be used to obtain 2 or 3 simultaneous views (left panels). The orientation of the scan planes (shown in the upper diagrams) can be modified by the operator during acquisition to obtain the desired view
The main technological breakthrough which allowed manufacturers to develop fully sampled matrix transducers has been the miniaturization of electronics that allowed the development of individual electrical interconnections for every piezoelectric element which could be independently controlled, both in transmission and in reception.
Beamforming (or spatial filtering) is a signal processing technique used to produce directionally or spatially selected signals sent or received from arrays of sensors. In 2DE, all the electronic components for the beamforming (high-voltage transmitters, low-noise receivers, analog-to-digital converter, digital controllers, digital delay lines) are inside the system and consume a lot of power (around 100 W and 1500 cm2 of personal computer electronics board area). If the same beamforming approach would have been used for matrix array transducers used in 3DE, it would have required around 4 kW power consumption and a huge PC board area to accommodate all the needed electronics to control 3000 piezoeletric elements. To reduce both power consumption and the size of the connecting cable, several miniaturized circuit boards are incorporated into the transducer, allowing partial beamforming to be performed in the probe (Fig. 1.3). This unique circuit design results in an active probe which allows microbeamforming of the signal with low power consumption (<1 W) and avoids to connect every piezoelectric element to the ultrasound machine. The 3000 channel circuit boards within the transducer controls the fine steering by delaying and summing signals within subsections of the matrix, known as patches (microbeamforming, Fig. 1.3). A typical patch for a cardiac matrix probe contains approximately 25 piezoelextric elements configured in 5 × 5 matrix. Only patches are connected to the mainframe beamformer within the system. This microbeamforming works like a very small electronic beamformer by pointing its 25 elements (on both transmit and receiving) toward the desired scan line and allows to reduce the number of the digital channels to be put into the cable that connects the probe to the ultrasound system from 3000 (which would make the cabling too heavy for practical use) to the conventional 128–256 allowing the same size of the 2D cable to be used with 3D probes. Each micro-beamformer has its own output, which is wired back to the ultrasound systemtrough the transducer cable. Coarse steering is controlled by the ultrasound system where the patches are time-aligned to produce the parallel receive beams for each transmit beam (Fig. 1.3).


Fig. 1.3
Three-dimensional beamforming. Beamforming with 3D matrix array transducers has been splitted in the transducer and the ultrasound machine levels. At the transducer level, interconnection technology and integrated analog circuits (DELAY) control transmit and receive signals using different subsection of the matrix (patches) to perform analog pre-beamforming and fine steering. Signals from each patch are summed to reduce the number of digital lines in the coaxial cable that connects the transducer to the ultrasound system from 3000 to the conventional 128–256 channels. At the ultrasound machine level, analog-to-digital (A/D) convertors amplify, filter and digitize the elements signals which are then focused (coarse steering) using digital delay (DELAY) circuitry and summed together (Ξ) to form the received signal from the desired object
However, the electronics inside the probe produce heat whose amount is directly proportional to mechanical index used during imaging, therefore the engineering of active 3DE transducer should include thermal management.
Finally, new and advanced crystal manufacturing processes allows production of single crystal materials with homogeneous solid state technology and unique piezoelectric properties. These new transducers result in reduced heating production by increasing the efficiency of the transduction process to improve the conversion of transmit power into ultrasound energy and of received ultrasound energy into electrical power. Increased efficiency of the transduction process together with a wider bandwidth result in increased ultrasound penetration and resolution which improve image quality with the additional benefits of reducing artifacts, lowering power consumption and increase Doppler sensitivity.
Further developments in transducer technology have resulted in a reduced transducer footprint, improved side-lobe suppression, increased sensitivity and penetration, and the implementation of harmonic capabilities that can be used for both gray-scale and contrast imaging. The last generation of matrix transducers are significantly smaller than the previous ones and the quality of 2D and 3D imaging has improved significantly, allowing a single transducer to acquire both 2D and 3DE studies, as well as of acquiring the whole LV cavity in a single beat.
3D echocardiography physics
3DE is an ultrasound technique and the physical limitation of the constant speed of ultrasounds in human body tissues (approximately 1540 m/s in myocardial tissue and blood) cannot be overcome. The speed of sound in human tissues divided by the distance a single pulse has to travel forth and back (determined by the image depth) results in the maximum number of pulses that can be fired each second without producing interferences. Based on the acquired pyramidal angular width and the desired beam spacing in each dimension (spatial resolution), this number is related to the volumes per second that can be imaged (temporal resolution). Therefore, similar to 2DE imaging, in 3DE imaging there is an inverse relationship between volume rate (temporal resolution), acquisition volume size and the number of scan lines (spatial resolution). Any increase in one of these factors will cause a decrease in the other two.
The relation between volume rate, number of parallel receive beams, sector width, depth, and line density can be described by the following equation:
therefore the volume rate can be adjusted to the specific needs by either changing the volume width or depth. The 3D system allows the user to control the lateral resolution by changing the density of the scan lines in the pyramidal sector too. However, a decrease in spatial resolution also affects the contrast of the image. Volume rate can also be increased by increasing the number of parallel receive beams, but in this way the signal-to-noise ratio and the image quality will be affected.

To put all this in perspective let us assume that we would like to image up to 16 cm depth in the body and acquire a 60° × 60° pyramidal volume. Since the speed of sound is approximately 1540 m/s and each pulse has to propagate 16 cm × 2 (to go forth and come back to the transducer), 1540/0.32 = 4812 pulses can be fired per second without getting interference between the pulses. Assuming that 1° beam spacing in both X and Z dimension is a sufficient spatial resolution we would need 3600 beams (60 × 60) to spatially resolve the 60° × 60° pyramidal volume. As a result, we will get a temporal resolution (volume rate) of 4812/3600 = 1.3 Hz, which is practically useless in clinical echocardiography.
The example above shows that the fixed speed of sound in body tissues has been a major challenge to the development of 3DE imaging. Manufacturers have developed several techniques such as parallel receive beamforming, multibeat imaging and real-time zoom acquisition to cope with this challenge but, in practice, this is usually achieved by selecting the appropriate acquisition modality for different imaging purposes (see Image acquisition and display paragraph).
Parallel receive beamforming or multiline acquisition is a technique where the system transmits one wide beam and receives multiple narrow beams formed within the bounds of the trasmit beam. In this way the volume rate (temporal resolution) is increased by a factor equal to the number of the received beams. Each beamformer focuses along a slightly different direction that was insonated by the broad transmit pulse. As an example, to obtain a 90° × 90°, 16-cm depth pyramidal volume at 25 vps, the system needs to receive 200,000 lines/s. Since the emission rate is around 5000 pulsed/s, the system should receive 42 beams in parallel for each emitted pulse. However, increasing the number of parallel beams to increase temporal resolution leads to an increase in size, costs and power consumption of the beamforming electronics, and deterioration in the signal-to-noise ratio and contrast resolution. With this technique of processing the received data, multiple scan lines can be sampled in the amount of time a conventional scanner would take for a single line, at the expense of reduced signal strength and resolution, as the receive beam are steered farther and farther away from the center of the transmit beam (Fig. 1.4).


Fig. 1.4
Parallel receive beamforming. Schematic representation of the parallel receive or multiline beamforming technique receiving 16 (left panel) or 64 (central panel) beams (blue squares) for each broad transmit beam (red pyramid). The right panel shows the degradation of the power and resolution of the signal (from red maximal to bright yellow minimal) from the parallel receiving beams steered farther away from the center of the transmit beam
Another technique to increase the size of the pyramidal volume and maintain the volume rate (or the reverse, e.g. maintain volume rate and increase the pyramidal volume) is the multibeat acquisition. With this technique, a number of small, ECG-gated subvolumes acquired from consecutive cardiac cycles are stitched together to build up the final, large pyramidal volume (Fig. 1.5). Multibeat acquisition will be effective only if the subvolumes to stitch together will be constant in position and size, therefore any transducer movement, cardiac translation motion due to respiration, change in cardiac cycle length will create subvolume malalignment and stitching artifacts (Fig. 1.6).



Fig. 1.5
Multibeat acquisition. Schematic drawing of a 4-beat full-volume acquisition. The 4 pyramidal subvolumes (the colors show the relationships between the pyramidal subvolumes, the ECG beats and the way the 3D data set has been built up in the lower part of the figure) are obtained from consecutive heart beats and are stitched together to build up the final full volume (blue square in the right lower part of the figure)

Fig. 1.6
Stitching artifacts. Volume-rendered image of the left ventricle displayed with respiratory gating artifacts. The blue lines highlight the misalignment of the pyramidal subvolumes
Finally, the quality of the images of the cardiac structures which can be obtained by a 3DE system will be affected by the point spread function of the system. The point spread function describes the imaging system response to a point input. A point input, represented as a single pixel in the “ideal” image, will be reproduced as something other than a single pixel in the “real” image (Fig. 1.7). The degree of spreading (blurring) of any point object varies according to the dimension employed. In current 3DE systems it will be around 0.5 mm in the axial (y) dimension, around 2.5 mm in the lateral (x) dimension, and around 3 mm in the elevation (z) dimension. As a result we will obtain the best images (less degree of blurring, i.e. distortion) when using the axial dimension and the worst (greatest degree of spreading) when we use the elevation dimension.


Fig. 1.7
Point spread function. (a) Graphical representation of the extent of distortion of a point passing through a optical system. (b) Effect of the point spread function on the final image of a circular object
These concepts have an immediate practical application in the choice of the best approach to image a cardiac structure. According to the point spread function of 3DE the best results is expected to be obtained by using the parasternal approach because structures are mostly imaged by the axial and lateral dimensions. Conversely, the worst result is expected to be obtained by the apical approach which mostly uses the lateral and elevation dimensions.
History of 3D Echocardiography
Since the early days of cardiovascular imaging, the concept of 3D imaging was indisputably perceived as a need based on the recognition that depicting the complex shape of the cardiovascular system in less than three dimensions severely limited the diagnostic value of the information obtained from medical imaging (Fig. 1.8). During the last half of the twentieth century, we have witnessed an impressive technological progress driven by strong demand from the cardiology community that moved from fuzzy single-projection X-ray films to multi-slices high spatial resolution tomographic images depicting anatomical details previously seen only in anatomy atlases. The possibility to visualize these details in living patients changed completely the way cardiologists understand disease processes and resulted in new standards in the diagnosis of diseases and patients’ management. Currently, both the diagnosis and treatment of most cardiovascular disease states heavily relies on information obtained by noninvasive imaging.


Fig. 1.8
Progress in ultrasound technology. From the early M-mode (1D), when one single ultrasound beam produced a time-depth display of cardiac structures, through two-dimensional echocardiography (2D), when a real-time tomographic display of the beating heart was made available, to current three-dimensional technology (3D), with which a real-time pyramidal acquisition of data is available
However, 3D imaging of the beating heart remained a challenge because of the constant motion of this organ. While 3D imaging of stationary organs was conceptually easy to solve by collecting information from different parts or from different angles consecutively, imaging of the beating heart required data collection to occur virtually in real time. This was a real challenge for engineers and physicists. In fact, it can be read in textbooks from the early 1970s explanations why real-time two-dimensional (2D) imaging of the beating heart is an enormous technological advancement that was believed to be unsurpasssable because of the limitations imposed by the constant speed at which ultrasound waves travel inside the human body.
Neverteless, despite the fact that the speed of sound has not changed since then, the combination of the exponential rise of computational power of computers with ingenious engineering solutions that increased the efficiency of the process of image formation from ultrasound reflections has meant that, during the last decade, 3DE has completed its transition from a predominant research tool to an imaging technique employed in everyday clinical practice [1].
This transition began in 2002 with the release of a reasonable user-friendly version of a matrix array transducer capable of real-time 3D imaging together with software which allows rapid slicing and quantification of 3DE data sets.
Before these technological achievements, attempts to develop 3DE imaging had relied in using a 2DE transducer (either tracking it in space or moving it in a prespecified pattern) to acquire multiple 2D views. This required spatial information about the ultrasound probe itself with the aid of cardiac and, in some technological solutions, respiratory gating to be assembled into a 3D reconstructed image using dedicated software. The ultrasound probe has been tracked via a variety of means either extrinsic and intrinsic to the probe. More recent systems obviate the need for these spatial tracking methods (Fig. 1.9).


Fig. 1.9
Temporal evolution of three-dimensional echocardiography technology (see text for details). Abbreviations: RT3DE real-time three-dimensional echocardiography, TEE transesophageal, TT transthoracic
The first attempt in imaging the human heart in 3D by ultrasound was made by Dekker et al. [7] in 1974, who used a mechanical articulated arm that measured probe displacement during the acquisition of multiple 2DE views to perform freehand transthoracic scanning (Fig. 1.10). This marked the birth of static surface rendering, the earliest 3D echocardiographic technique. This work demonstrated the possibility of producing a 3D data set, but the technology was impractical for clinical use.


Fig. 1.10
The mechanical arm. Schematic drawing of the scanning bed and the device (left panel) and actual picture of the arm with the probe (right panel). A large external beam device gives spatial data regarding an ultrasound probe to enable three-dimensional reconstruction. The ultrasound probe is highlighted by a red circle
Trying to improve the tracking method, Moritz and Shreve [8] developed an acoustic locator (the so called “spark-gap) whereby an acoustic element was attached to the ultrasoud probe and sent regular audio pulses that were detected by a fixed antenna to be included in a Cartesian locator grid (Fig. 1.11).


Fig. 1.11
Acoustic locator or “spar-gaps”. The ultrasound probe position is tracked by a means similar to radar technology
In 1977, Raab et al. [9] reported about an electromagnetic sensor which could be attached to the ultrasound probe allowing continuous monitoring of its position in space. Although this technique was quite advanced for 1977, it was not used systematically until the mid 1990s (Fig. 1.12). Several other Authors published early attempts to produce 3DE data sets during this time period [10–12]. Further development of these techniques led to what has been termed “free-hand scanning”. With this modality, a highly developed magnetic-field system orients sequentially acquired 2DE imaging planes by tracking the movement of the ultrasound probe as it is manipulated by the operator.


Fig. 1.12
Freehand scanning. A modified ultrasound probe is tracked in 3D space using an electromagnetic locator system (left panel). Schematic drawing of the receiver and transmitting device and the Cartesian coordinate system for tracking the location of the transducer (right panel). Images then may be reconstructed off-line to create 3D data sets
One crucial developmental aspect for the success of 3D reconstruction from multiplane acquisition was the registration of the different planes, so that they could be combined together to create a 3D image of the heart. This was achieved by sequential gated acquisition, wherein different cut planes were acquired one-by-one with gating designed to minimize artifacts. To minimize spatial misalignment of slices because of respiration, respiratory gating was used, such that only cardiac cycles coinciding with a certain phase of the respiratory cycle were captured. Similarly, to minimize temporal misalignment because of heart rate variability, ECG gating was used, such that only cardiac cycles within preset limits of R-R interval were included. This methodology became standard in both transthoracic and transesophageal multiplane imaging aimed at 3D reconstruction and was widely used until real-time 3D imaging became possible.
To achieve effective multiplane acquisition, new techniques were developed that operated under the assumption that both the patient and the housing of the ultrasound probe remained in a relatively fixed position. The ultrasound probe was held by a device that moved the probe in a specified, preprogrammed fashion within the housing of a mechanical device. Examples were linear, fan-like and rotational acquisition methods (Fig. 1.13).


Fig. 1.13
Gated sequential imaging. Transducer motion modalities to generate 3DE images using gated sequential imaging. Linear (left panel): multiple parallel equi-distantly spaced 2D images can be obtained by progressive computer-controlled linear motion of the transducer in prespecified depths. Fan-like (central panel): multiple two-dimensional images are obtained in a fan-like array by tilting the transducer in specified arc angles to create a pyramidal data set. Rotational (right panel): multiple two-dimensional images are obtained by rotating the transducer 180° and obtaining images at pedetermined intervals (e.g. every 3° or 6°) to create a conical data set
In the early 1990s, the use of linear step-by-step motion acquisition, wherein the transducer was mechanically advanced between acquisitions using a motorized driving device become commercially available [13].
However, this simple solution was not applicable for transthoracic echocardiography, because of the need to find intercostal acoustic windows for each acquisition step. This approach was implemented in a pull-back transesophageal echocardiography transducer, known as “lobster tail” probe (Fig. 1.14, Right panel). In the handle of the transducer there was a motorized unit that incrementally pulled crystals in a parallel fashion at 1 mm increments gated to electrocardiogram (ECG) and respiration. The first transesophageal 3DE study was performed in the early 90s using this device [14].


Fig. 1.14
Motorized linear-motion device used to acquire parallel cut planes for reconstructing 3D images using linear step-by-step transducer motion (left panel). Pull back transoesophageal probe that employed the same approach of linear motion (right panel)
An alternative approach to linear scanning was to keep the transducer in a fixed position corresponding to an optimal acoustic window, and rotate the imaging plane by internally steering the imaging element in different directions (Fig. 1.15). This concept of rotational scanning in combination with gated sequential scanning was implemented into transoesophageal technology and resulted in a probe that has subsequently become the main source of multiplane images used for 3D reconstruction, both for research and clinical practice [15, 16].


Fig. 1.15
Rotational approach implemented into a transoesophageal multiplane transducer (left panel). Schematic drawing of sequential ultrasound images obtained by progressive rotation of the transducer in a prespecified fashion (central panel). Example of a 3D image of the mitral valve with anterior leaflet prolapse from multiplane images acquired using this transducer (right panel)
This approach provided 3D reconstructions of reasonably good quality due to the high quality of the original 2D images and the fact that the transoesophageal probe is relatively well “anchored” in its position throughout image acquisition, especially in sedated patients. Nevertheless, cardiac structures, such as valve leaflets appeared jagged as a result of stitch artifacts (Fig. 1.15, right panel), reflecting the contributions of individual imaging planes that could not be perfectly aligned during reconstruction, despite the ECG and respiratory gating. Multiple studies demonstrated the clinical usefulness of this approach mostly in the context of the evaluation of valvular heart disease.
An early transthoracic implementation of rotational approach consisted of a motorized device that contained a conventional transducer (Fig. 1.16), which was mechanically rotated several degrees at a time, resulting in first transthoracic gated sequential multiplane acquisitions suitable for 3D reconstruction of the heart [17–19].


Fig. 1.16
Motorized device that housed an external stepper motor that mechanically rotated a built-in transthoracic transducer.
Despite the previously unseen transthoracic 3DE images that excited so many, it quickly became clear that this methodology was destined to remain limited to the research arena because image acquisition was too time-consuming and tedious for clinical use. In addition, the quality of the reconstructed images was limited.
With each of these methods, once the numerous 2D “planes” have been obtained according to prescribed transducer movements, the images had to be realigned and digitally reformatted into rectangular pixels that were then stacked. Gaps between adjacent images were filled by interpolation. A cubic volume of “voxels” was then derived from the stacked images that could then be volume rendered or “sliced” in any plane of interest (Fig. 1.17).


Fig. 1.17
Reconstruction of a 3D data set from multiple 2D images. The collection of images (typically 15 to 60 cardiac cycles, with 10 to 15 frames/heart beat) were re-sampled in a cubical data set and ultimately rendered into a 3D image or sliced according to planes of interest
A profound limitation to each of these techniques was that they fundamentally relied on the acquisition of multiple 2D images. Image quality was therefore highly dependent on concordance between adjacent images. The inherent need to interpolate data became problematic if the adjacent images were themselves discordant due to voluntary or involuntary patient movement. Problems inherent to irregular heart rate and lung artifact were minimized by using cardiac and respiratory gating to prespecified heart rates and phases of the respiratory cycle. Images were therefore acquired over a varying period of time, often up to 2–3 min, and even longer in the presence of atrial fibrillation. This was because, from a practical standpoint, the image mismatch was too profound if the R-R gating was set to vary by more than roughly 150 ms. If a patient with atrial fibrillation was gated at 150-ms variation, the acquisition could take up to 10 min. In this setting, it was unlikely that either the patient or the physician obtaining the data could remain stationary for this length of time. Actually, to obtain a satisfactory 3D reconstruction with this technology, it was required a very skilled operator, a very cooperative patient, an operator skilled with 3D reconstruction and a bit of luck. If succesful, although image quality was suboptimal for small discrete structure like valve morphology analysis, even at this time, data sets were useful for ventricular volume assessment.
However, the presence of the locator device, which, limited significantly the portability of the ultrasound system, the need of avoiding any metallic close to the system and the patient, the long reconstrunction times and the unpredictability of the final results have further hindered more widespread use of this technique in clinical practice.
The collective experience and the limitations of the gated sequential acquisition and offline 3D reconstruction gradually led developers to the understanding that scanning volumes rather than isolated cut planes would intrinsically address many of the previous issues. This revolutionary idea led von Ramm et al. [20, 21] to develop the first real-time 3DE system at Duke university. This system was equipped with a phased-array transducer, in which piezoelectric elements were arranged in multiple rows, rather than one row, allowing fast sequential scanning of multiple planes. The phased array technology, that has been an integral part of the 2D transducers for decades, was modified to electronically change the direction of the beam not only within a single plane to create a fan-shaped scan, but also in the lateral direction to generate a series of such scans. Importantly this was achieved without any mechanical motion, allowing the speeds necessary for volumetric real-time imaging.
The first generation of real-time 3D transducers was bulky due to unprecedented number of electrical connections to the individual crystals, despite the relatively small number of elements in each row. This sparse array matrix transducer consisted of 256 non-simultaneously firing elements and had large footprint, which did not allow good coupling with the chest wall for optimal acoustic windows, and produced 3D images that were suboptimal in any selected plane, when compared to the quality of standard at the time 2D echocardiographic images. Nevertheless, the mere fact of successful real-time 3D imaging of an entire volume of heart using one cardiac cycle was a huge technological breakthrough.
This represented a major transition from the thin slice sector imaging obtainable with 2DE. Despite volumetric acquisition was a very positive development, the images were displayed as 2D (Fig. 1.18). The 2D images were derived from the 3D data set but were displayed as 2D orthogonal cut planes.


Fig. 1.18
The first real-time 3D echocardiography system (C-scan, Volumetrics, Inc.) equipped with a sparse matrix array transducer (left panel). In the right panel the images which can be obtained with that system are shown: apical views of the heart on the right (4-chamber on top, and 2-chamber of the left ventricle on the bottom), and short axis, or C scans, of the LV derived from perpendicular cuts through the apical views on the left
However, the road was paved and in November 2002, at the time of the American Heart Association meeting, Philips released the first generation of real-time 3DE.
Technical Features and Limitations of 3D Echocardiography
By being a tomographic technique, conventional 2DE relies heavily on the operator’s experience to “mentally” reconstruct the complex shape of cardiac structures from a limited number of “slices” (Fig. 1.19). As such, 2DE is limited in its ability to illustrate complex cardiac strucural relationships and relies on assumptions about image plane position and cardiac structure geometry when quantitation is performed.


Fig. 1.19
Mental reconstruction of complex cardiac structure from a limited number of tomographic views obtained with conventional two-dimensional echocardiography. Since no single view represents the actual 3D shape of the mitral valve, the echocardiographer acquires a number of 2DE views from different approaches and then start a mental process to integrate these views to obtain a stereoscopic representation of the actual valve. It is evident how this process (and the results) are affected by the operator experience and his/her practice of the anatomical theatre and/or operating room
3DE is a volumetric technique which allows an “electronic” cutting of the data set (similar to what is done in the anatomical theatre by the anatomist) to provides a virtually unlimited sections of the heart and its structures. Compared to conventional 2DE, the operator can obtain unique views of the heart (e.g. the “surgeon view”of the cardiac, like the mitral or the tricuspid valve seen from the atrium or the aorta seen from the aortic root) and perform quantitative analyses of the geometry and function of cardiac structures without making any assumption about their shape. As an example, if we want to use 2DE to calculate the volumes of the LV we can use different formulas (Fig. 1.20) which assume that the LV has a certain shape that can be assimilated to a known geometrical figure and the volume is calculated from simple actual measurements (usually areas and linear dimensions). With 3DE, there is no calculation to be performed. The endocardium of the LV is mapped to create a beutel whch represent the cardiac cavity (Fig. 1.21) and the volume is measured by simply counting the voxels within the beutel. The ability to acquire volumetric data sets which contains the whole cardiac cavity and to measure volumes without the need of geometrical assumptions have prompted the possibility of measuring the volumes of geometrically complex structures like the right ventricle which are impossible to calculate with 2DE [22].



Fig. 1.20
Schematic representation of the main geometric assumptions used to calculate left ventricular (LV) volumes by M-mode and two-dimensional echocardiography, and their correspondence to actual shape of distorted ventricle (apical aneurysm). A1, LV short-axis area at mitral valve level, A2, LV short-axis area at papillary muscle level, L length, LAX LV long axis, LVID LV internal diameter

Fig. 1.21
Three-dimensional measurement of left ventricular volume. Volume rendering of a three-dimensional multibeat acquisition focused on the left ventricle (left panel). The data set is segmented in 3 longitudinal (apical 4- and 2-chamber and long-axis) views and 1 transversal view (SAX) (Right panel). The three longitudinal views share the same apex (as it can be seen on the SAX) and their position can be adjusted by rotating the yellow (4CH), purple (3CH) and green (2CH) lines in order to achieve proper view orientation. Semi- or fully-automated tracing of the endocardial border in these views allows a mapping of the entire endocardial surface which is rendered in the surface rendering beutel (right lower angle of the right panel). The count of the voxels in the beutel at different moments of the cardiac cycle will provide the measurement of the LV volumes
Accordingly, the clinical usefulness of 3DE has been shown in several areas, including: (i) measurement of cardiac chamber volumes without the need of geometric modeling and without the detrimental effect of foreshortened views [3, 22–25]; (ii) unique, anatomically sound and realistic views of cardiac valves and congenital abnormalities [26–28]. This has been proven to be extremely useful to understand the pathophysiology and quantitate the severity of the diseases and to guide and assess effectiveness of surgical and/or percutaneous transcatheter interventions [29]; (iii) Visualization of regional wall motion [30]; (iv) Localization and visualization regurgitant or shunting jets with 3D color Doppler. In some instances, the scientific evidence seems strong enough to endorse the use of 3DE as a new standard in the clinical assessment of the heart [31].
However, despite 3DE clearly offers new possibilities to acquire, display and quantify cardiac structures, current 3DE systems are not yet able to fully replace conventional high-end 2DE systems. The main shortcomings in currently available 3D echo technology are: (i) 3DE systems are technically demanding to be properly used for both data set acquisition and post-processing; (ii) both temporal and spatial resolution are suboptimal (particularly in single beat acquisition mode); (iii) Software tools for quantitative analysis of 3D echo data sets are limited (currently there is no software package to quantitate the atria and the tricuspid valve) and most of those which are available works only with vendor specific data sets; and iv. lack of a DICOM standard to quantify and crop the 3D echo data sets.
For effective application of the 3DE technique, echocardiographers need specific education and training. They have to learn how to acquire volumetric data sets without artifacts, and navigate within the data set to obtain the desired cut plane or extract the needed views. New tools like cropping, slicing and thresholding are available to manipulate the data sets in order to visualize the cardiac structure of interest. Various ways to display the information are available (see next paragraph). Compared with the reassuring simplicity of obtaining 2DE imaging which is virtually the same on every echocardiographic machine, the workflow for both 3D data sets acquisition and post-processing, which is specific for each company, definitely needs to be standardized and simplified.
Theoretically, spatial and temporal resolution of 3DE data sets can be similar or even higher than the one of 2DE images. This can be obtained by using a much larger number of piezoelectric crystals in the probe. All of them should be controlled independently during both the transmitting and receiving process, more efficient beam forming techniques need to be implemented, and the data should be transferred in real-time to the system to build up the final data sets. However, this implies problems of miniaturization, probe heating management, huge data streams and complex calculations that cannot be implemented in the current generation of echocardiographic systems. Several research groups have the equipment to obtain high resolution 3DE data sets, but it requires long acquisition times, off line processing and the reconstruction of a data set from a single heart beat may require hours or days. However, new materials are continuously created to improve the efficience of crystals, and the computational power of computers is growing exponential, therefore it can be expected that future echocardiographic systems will be able to handle this data and provide high frame rate 3DE imaging.
Acquiring 3DE data sets of the different cardiac structures is feasible however, availability of tools able to allow advanced 3D quantitation, display and printing of cardiac structures is relatively limited. In particular, there is a definite need of developing new software packages to assess tricuspid valve, atria, RV shape and strain and also improve the robustness and accuracy of automated measurement software packages in order to increase feasibility and reproducibility of measurements.
Modalities of Displaying 3D Imaging
Currently, 3D data set acquisition can be easily implemented into standard echocardiographic examination by either switching among 2D and 3D probes or, with newest all-in-one-probes, by switching between 2D and 3D modalities available in the same probe. The latter probes are also capable to provide single-beat full-volume acquisition, as well as real-time 3D color Doppler imaging.
At present three different methods for 3D data set acquisition are available [5]:
multiplane imaging
“real-time” (or “live”) 3D imaging
multi-beat ECG-gated imaging
In the multiplane mode, multiple, simultaneous 2D views can be acquired at high frame rate using predefined or user-selected plane orientations and displayed using the split screen option (Fig. 1.2). The first view on the left is usually the reference plane that is orientated by adjusting the probe position while the other views represents views obtained from the reference view by simply tilting and/or rotating the imaging planes. Multiplane imaging is a real time acquisition and secondary imaging planes can only be selected during acquisition. Doppler colour flow can be superimposed on 2D images and in some systems both tissue Doppler and speckle tracking analysis can be performed. Although strictly not a 3D acquisition, this imaging mode is useful in situations where assessment of multiple views from the same cardiac cycle is desirable (e.g. atrial fibrillation or other irregular arrhythmias, stress echo, evaluation of interventricular dyssynchrony, analysis of regurgitant jet extension and shape, etc.)
In the real-time mode, a pyramidal 3D volumetric data set is obtained from each cardiac cycle and visualized live, beat after beat as during 2D scanning. As the data set is updated in real-time, data set orientation and cut plane can be changed by simply rotating or tilting the probe. Visualization of cardiac structures is obtained with limited post-processing and the data set can be rotated (independent of the transducer position) to view the cardiac structure of interest from different orientations. Heart dynamics is shown in a realistic way, with instantaneous on-line volume rendered reconstruction. Real-time modality allows fast acquisition of dynamic pyramidal data structures from a single acoustic view that may encompass the entire heart without the need of reference system, electrocardiographic (ECG) and respiratory gating. Real-time imaging is time-saving both for data acquisition and analysis. Although this acquisition mode overcomes rhythm disturbances or respiratory motion limitations, it still suffers of relatively low temporal and spatial resolution. Real-time imaging can be acquired in the following modes:
- (a)
Live 3D. Once the desired cardiac structure has been imaged in 2DE it can be converted to a volumetric image by pressing a specific button in the control panel. The 3D system automatically switch to a narrow sector acquisition (approximately 30° × 60° pyramidal volume) to preserve spatial and temporal resolution. The size of the pyramidal volume can be increased to visualize larger structures, but both scan line density (spatial resolution) and volume rate (temporal resolution) will drop down. 3D live imaging mode is used to: (i) guide full-volume acquisition; (ii) Visualize small structures using the zoom mode (aortic valve, masses etc); (iii) Recording short-lived events (i.e. bubble passage); (iv) in patients with irregular rhythm/dyspnea unable to cooperate for a full-volume acquisition; (v) guiding/monitoring interventional procedures.
- (b)
Live 3D colour. Colour flow can be superimposed on a live 3D data set to visualize blood flow in real time. With this modality, temporal resolution is usually very low
- (c)
3D zoom. This imaging mode is an extension of live 3D and allows a focussed real time view of a structure of interest. A crop box is placed on a 2D single- or multiplane image to allow the operator to adjust lateral and elevation width to include the structure of interest in the final data set, then the system automatically crops the adjacent structures to provide a real time display of the structure of interest with high spatial and temporal resolution. The draw-back of the 3D zoom mode is that the operator loses the relationships of the cardiac structure of interest with surrounding structures. This acquisition modality is mainly used during transoesophageal studies for detailed anatomical analysis of the structure of interest
- (d)
Full-volume. The full-volume provides has the largest acquisition volume possible (usually 90° × 90° degrees). Real-time (or “single-beat”) full-volume acquisition is affected by low spatial and temporal resolution and it is used for quantification of cardiac chambers when multibeat ECG gated acquisition is not possible (e.g. irregular cardiac rhythm, patient unable to cooperate for breath-holding)Stay updated, free articles. Join our Telegram channel
Full access? Get Clinical Tree
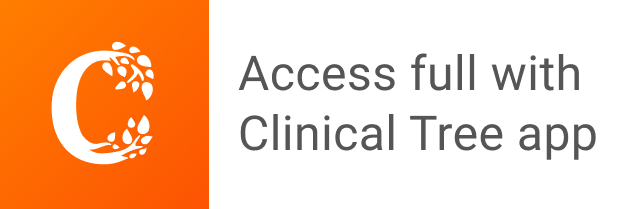