Abstract
The triad of preload, afterload, and inotropic state of the heart determines stroke volume, which together with heart rate determines cardiac output. The parasympathetic and sympathetic nervous systems regulate cardiac inotropy (contractility), chronotropy (heart rate), and lusitropy (myocardial relaxation). An understanding of the anatomy, physiology, and molecular biology of the sympathetic and parasympathetic nervous systems forms the basis of pharmacologic manipulation of the cardiovascular system. This chapter presents basic principles and clinical applications of medicinal therapies used to optimize cardiac output and systemic perfusion.
Key Words
Pharmacology, Adrenoreceptors, Inotropic drugs, Beta-blockers, Vasodilation, Vasoconstrictor
The triad of preload, afterload, and inotropic state of the heart determines stroke volume, which together with heart rate, determines cardiac output. The parasympathetic and sympathetic nervous systems regulate cardiac inotropy (contractility), chronotropy (heart rate), and lusitropy (myocardial relaxation). An understanding of the anatomy, physiology, and molecular biology of the sympathetic and parasympathetic nervous systems forms the basis of pharmacologic manipulation of the cardiovascular system. This chapter presents basic principles and clinical applications of medicinal therapies used to optimize cardiac output and systemic perfusion. Antiarrhythmic drugs are presented in Chapter 27 . Medications for treatment of pulmonary hypertension are presented in Chapter 71 .
Autonomic Control of the Cardiovascular System
Sympathetic (Adrenergic) Control
Sympathetic Neuroanatomy.
Autonomic control of the cardiovascular system is determined by the sympathetic and parasympathetic inputs. The cell bodies of the preganglionic sympathetic neurons are located in the anterolateral gray matter of the spinal cord from T1 to L3. Preganglionic axons synapse with postganglionic neurons at sympathetic ganglia, which are collected into two paravertebral chains, three abdominal prevertebral ganglia (celiac, superior mesenteric, and inferior mesenteric ganglia), and left and right cervical ganglia (inferior, middle, and superior cervical ganglia) ( Fig. 19.1 ).

The sympathetic nervous system also directly innervates the adrenal gland and controls the release of the catecholamines epinephrine and norepinephrine. Innervation occurs via preganglionic fibers that travel through the sympathetic chain, exit via the splanchnic nerve, pass through the celiac ganglion without synapsing, and directly innervate the adrenal medullary cells. During sympathetic stimulation, acetylcholine is released by the preganglionic fibers, resulting in the opening of calcium-mediated channels on the cell membranes. These calcium channels then modulate the exocytosis of granules containing epinephrine and norepinephrine. Epinephrine and norepinephrine then travel through the systemic circulation, where they act on adrenoreceptors throughout the body.
Adrenoreceptors.
Endogenous neurohormones (e.g., epinephrine and norepinephrine) and exogenous adrenergic drugs exert their cardiovascular effects by combining with adrenergic receptors in the heart and vasculature. Although genes for at least nine distinct adrenoreceptor subclasses have been identified and cloned, the clinical pharmacology of adrenergic drugs is still largely based on the four classic receptor subtypes: alpha 1 , alpha 2 , beta 1 , and beta 2 . Norepinephrine and epinephrine are agonists for alpha and beta receptors in the vasculature and the myocardium. Myocardial adrenergic receptors are primarily (80% to 85%) of the beta subclass. Stimulation of the myocardial beta receptor leads to increased sinus node firing rate, more rapid atrioventricular conduction, and increased myocardial contractility. Alpha 1 receptors constitute a minority of the total adrenergic receptors in ventricular myocardium. Stimulation of alpha 1 receptors also has a positive inotropic effect. The central cellular mechanism underlying increased myocardial contractility is an increase in intracellular Ca 2+ concentration in response to stimulation of adrenergic receptors in the sarcolemma. This process is initiated and amplified by a membrane-bound transduction system consisting of adrenergic receptors, guanine nucleotide-binding proteins (G proteins), and the effector enzyme adenyl cyclase.
Vascular smooth muscle contains mainly beta 2 and alpha 1 receptors with beta 2 agonism leading to vasodilation and alpha 1 agonism to vasoconstriction. Norepinephrine has only a small effect on beta 2 receptors but is a potent alpha 1 agonist leading to intense vasoconstriction. Conversely, low concentrations of epinephrine are sufficient to stimulate beta 2 receptors, whereas higher epinephrine concentrations are required for alpha 1 receptor stimulation.
Alpha 2 receptors are found primarily in preganglionic and postganglionic sympathetic nerve terminals to peripheral vasculature and within the central nervous system (CNS). Whereas stimulation of postganglionic alpha 2 receptors leads to vasoconstriction, the overall effect of an alpha 2 agonist such as clonidine is sympathetic inhibition because norepinephrine secretion is inhibited from the preganglionic nerve terminal and/or sympathetic outflow is decreased from the CNS.
Myocardial Beta 1 and Beta 2 Receptors.
Myocardial adrenergic receptors in the nonfailing heart are 65% beta 1 and 20% beta 2 . Norepinephrine, the dominant endogenous cardiac neurotransmitter, has 30 to 50 times greater affinity for the beta 1 compared with the beta 2 receptor. Thus in the nonfailing heart, beta 1 is the predominant regulator of contractility and heart rate. Activation of myocardial beta 1 and beta 2 receptors results in increased inotropy and chronotropy. The different adrenoreceptor subclasses use different G proteins and second messengers. In the case of the beta receptor the major events in this process consist of a first messenger (e.g., epinephrine) binding to a beta 1 or beta 2 receptor, which produces a conformational change in the receptor so that it can activate the stimulatory G protein (Gsα) ( Fig. 19.2 ). The G protein functions as a transducer of the signal by activating the effector enzyme adenyl cyclase. Activation of adenyl cyclase amplifies the signal through the production of the second messenger, cyclic adenosine monophosphate (cAMP). Finally, cAMP activates protein kinase A, leading to phosphorylation of a variety of cAMP-dependent proteins, which ultimately effect excitation-contraction coupling. Because cAMP is broken down by specific phosphodiesterase, inhibition of phosphodiesterase raises cAMP levels and increases myocardial contractility (see section on phosphodiesterase inhibitors).

Among the proteins activated by protein kinase A is the L-type, voltage-sensitive calcium channel in the sarcolemmal membrane. Activation of this calcium channel allows entry of a small amount of extracellular calcium into the cell, which binds to the ryanodine receptor (RyR2) on the sarcoplasmic reticulum. Binding of calcium to the ryanodine receptor triggers the release of micromolar amounts of calcium from sarcoplasmic reticulum into the cytoplasm. This process is known as calcium-induced calcium release, which initiates contraction by binding to the troponin complex, a series of regulatory proteins on the actin molecule. The conformational changes within the troponin complex reverse the inhibition of actin and myosin, thereby allowing contraction to take place. Relaxation of the myocardial fiber becomes possible when reuptake of cytosolic calcium into sarcoplasmic reticulum occurs via energy-dependent sarcoplasmic reticulum/endoplasmic reticulum calcium adenosinetriphosphatase (ATPase) (SERCA) pumps. Any drug that improves myocardial fiber contractility must either increase the concentration of intracellular calcium or increase the sensitivity of the myocardial fiber to calcium.
Vascular Beta 2 Receptors.
Beta 2 -adrenergic receptors in the vascular smooth muscle mediate dilation and relaxation. Activation of the beta 2 vascular receptor similarly results in increased intracellular cAMP. However, here cAMP inhibits myosin light chain kinase, resulting in vascular smooth muscle relaxation ( Fig. 19.3 ).

Myocardial and Vascular Alpha Receptors.
Alpha 1 receptors constitute 13% to 15% of the adrenergic receptors in ventricular myocardium. Once an alpha 1 agonist binds to the alpha 1 receptor, a different G protein (G αq ) is activated and, in turn, activates phospholipase C (see Fig. 19.2 ). The activated phospholipase C hydrolyzes membrane-bound phospholipids to inositol triphosphate (IP 3 ) and diacylglycerol (DAG). IP 3 stimulates the release of Ca 2+ from sarcoplasmic reticulum. DAG activates protein kinase C and subsequent phosphorylation of intracellular proteins, which regulate cell responsiveness.
Adrenoreceptors in Heart Failure.
Circulating levels of norepinephrine are raised in heart failure. There is a selective downregulation in the number of myocardial beta 1 receptors without a change in the number of beta 2 or alpha 1 receptors, resulting in an increased percentage of the latter two receptors (47% beta 1 , 25% beta 2 , and 28% alpha 1 ). Either increased receptor destruction or decreased receptor synthesis (or both) may cause downregulation. In addition, uncoupling of the beta 2 receptor from the G protein–adenylate cyclase complex prevents effective signal transduction. Another proposed mechanism of beta-receptor desensitization involves sequestration or internalization of the receptor away from the cell surface. In contrast to downregulation, sequestration permits recycling the receptor back to the cell surface once chronic beta agonist exposure has ceased.
Cardiopulmonary bypass (CPB) with aortic cross-clamping is a potent stimulus for release of myocardial norepinephrine. This could explain myocardial beta-adrenergic desensitization observed in children following CPB with aortic cross-clamping, which is postulated to result from beta-receptor uncoupling.
As a result of beta 1 downregulation and beta 2 uncoupling, inotropic drugs fail to yield previously attainable levels of hemodynamic support in conditions of heart failure or following CPB with aortic cross-clamp.
Adrenoreceptors and Exogenous Agonists.
Similar to chronic exposure to elevated endogenous norepinephrine levels in heart failure, chronic administration of exogenous agonists induces downregulation of beta receptors. Animal studies indicate that after 7 days of isoproterenol (beta 1 and beta 2 agonist), beta 2 receptors are decreased 60% to 80%, whereas beta 1 receptors remain unchanged.
Parasympathetic Control
The parasympathetic innervation to the heart is provided by the vagus nerve. The long preganglionic fibers synapse in ganglia within the heart from which very short postganglionic fibers travel to the sinus node, atrioventricular node, and cardiomyocytes. Acetylcholine is the neurotransmitter released by both preganglionic and postganglionic parasympathetic nerve terminals (see Fig. 19.1 ). Myocardial muscarinic receptors are predominantly of the M2 subtype. Vagal (cholinergic) stimulation depresses cardiac rate and contractile function by limiting the accumulation of intracellular Ca 2+ . These negative inotropic effects are mediated by activation of inhibitory receptors in the sarcolemma.
Muscarinic receptor density is 2- to 2.5-fold greater in the atria than in the ventricles in contrast to the uniform distribution of beta receptors. Muscarinic receptors are bound to an inhibitory G protein. Activation of this receptor leads to inhibition of adenylate cyclase activity and the subsequent decrease in intracellular cAMP levels. Muscarinic agonists may also stimulate opening of K + channels in atrial pacemaker cells, leading to K + influx and hyperpolarization of the cell membrane. The latter mechanism may also be linked to G proteins and account for the bradycardic effect of muscarinic drugs.
Similarly, the transmembrane adenosine A 1 receptor is coupled to inhibitory G protein. Activation of the adenosine A 1 receptor in the myocardium decreases cardiac oxygen demand by opening sarcolemmal K + channels, resulting in decreased heart rate and decreased contractility. In addition, activation of adenosine A 1 receptors antagonizes beta-adrenergic stimulation by inhibiting the formation of cAMP by adenylate cyclase. Thus the adenosine A 1 receptor mediates the negative chronotropic, inotropic, and anti–beta-adrenergic effects of adenosine.
Transplanted Heart
In humans, cardiac beta 1 receptors may represent “innervated” receptors and are involved mainly in neuronal control of the heart. Beta 2 receptors may represent “hormonal” receptors, responding more to circulatory agonists, and mediate both chronotropic and inotropic responses. In healthy hearts of humans the proportion of beta 2 receptors in ventricular myocardium is approximately 20% and between 25% and 40% in atrial tissue. Hemodynamic responses to the endogenous catecholamines, norepinephrine and epinephrine, differ markedly between heart transplant patients and nontransplant patients with mild essential hypertension. Cardiac transplantation results in near complete sympathetic and parasympathetic denervation of the donor heart, which results in an altered hemodynamic response to beta-receptor stimulation. Beta-adrenergic stimulation of the transplanted heart results in increased heart rate because the denervation removes parasympathetic buffering that would otherwise be activated by the arterial baroreflex. For example, a transplanted heart may respond to exercise via increases in heart rate (as opposed to an inotropic response) that are largely related to increases in circulating endogenous catecholamines, secondary to release from the adrenal medulla. Heart rate responses to isoproterenol have been shown to be enhanced in cardiac transplant recipients compared with healthy subjects but not enhanced or decreased compared with healthy subjects treated with atropine, to exclude potential reflex negative inotropic changes in innervated hearts mediated by the parasympathetic nervous system. In addition to increased responsiveness to isoproterenol, the denervated heart also has increased responsiveness to dobutamine, both of which may reflect upregulation of beta-adrenergic receptors.
Inotropic Drugs
Dopamine
Dopamine is a sympathomimetic amine capable of directly stimulating beta 1 and alpha 1 receptors and works indirectly as an intermediary in the enzymatic pathway leading to the production of norepinephrine and epinephrine. Dopamine receptors are found in the coronary, renal, mesenteric, and cerebral arteries. Dopaminergic-1 receptors are postsynaptic and result in vasodilation. Dopaminergic-2 receptors are presynaptic and result in vasodilation due to inhibition of release of norepinephrine ( Table 19.1 ).
Drug | Dose | α 1 | β 1 | β 2 | Dopa | Usual Titration a | Cautions/Comments |
---|---|---|---|---|---|---|---|
Dobutamine | Usual: 2-20 mcg/kg/min | + | +++ | + | 0 | 1-2 mcg/kg/min every 10 min until desired effect is reached |
|
MAX: 30 mcg/kg/min | |||||||
Dopamine | <5 mcg/kg/min | 0 | ++ | 0 | ++++ | 1-5 mcg/kg/min every ≥5 minutes |
|
5-10 mcg/kg/min | ++ | +++ | + | ++ | 1-10 mcg/kg/min every ≥5 min | ||
>10 mcg/kg/min | ++++ | +++ | + | + | |||
Epinephrine | Usual: 0.01-1 mcg/kg/min | ++ | +++ | ++ | 0 | 0.01-0.1 mcg/kg/min every ≥1 min until desired effect is reached |
|
MAX: 2 mcg/kg/min | |||||||
Norepinephrine | Usual: 0.05-1 mcg/kg/min | ++++ | ++ | 0 | 0 | 0.01-0.1 mcg/kg/min every ≥2 min until desired effect is reached |
|
MAX: 2 mcg/kg/min | |||||||
Phenylephrine | Usual: 0.04-2 mcg/kg/min | ++++ | 0 | 0 | 0 | 0.05-0.3 mcg/kg/min every ≥5 min until desired effect is reached |
|
MAX: 4 mcg/kg/min |
a Titrations may vary based upon clinical indication and patient status.
The pharmacokinetics of dopamine in children is not clearly characterized due to the difficulty in differentiating endogenous versus exogenous concentrations. The pharmacokinetic data that exist suggest high interindividual variability. In a heterogeneous group of pediatric intensive care unit patients, the t 1/2 α, (distribution half-life) was 1.8 ± 1.1 minutes, and the t 1/2 β, (elimination half-life) was 26 ± 14 minutes compared with another study of newborns in which the t 1/2 β was 7 minutes. The impact on t 1/2 by liver or renal dysfunction in unknown because some studies have shown decreased clearance and others no change compared with individuals without hepatic or renal dysfnction. Thirty percent of plasma dopamine is protein bound. Dopamine is degraded by two enzyme systems: catechol-O-methyl transferase (COMT) and monoamine oxidase (MAO).
Concomitant administration of dobutamine may affect dopamine clearance. In the presence of dobutamine, dopamine clearance increases linearly as the dopamine infusion rate is raised.
Children younger than 2 years of age clear dopamine twice as fast as those older than 2 years. This may in part explain the fact that infants require a higher dopamine infusion rate to achieve an effect equivalent to that found in older children and adults.
The hemodynamic effects of dopamine are dose dependent, with renal vasodilation evident at low infusion rates of less than 5 mcg/kg/min, increased inotropy at moderate infusion rates of 5 to 10 mcg/kg/min, and increased vascular resistance at higher infusion rates, greater than 10 mcg/kg/min (see Table 19.1 ). There is little, if any, cardiovascular improvement with lower doses of dopamine (<5 mcg/kg/min), but cardiac index has been shown to increase by 17%, with a concomitant 70% rise in renal plasma flow with doses greater than or equal to 5 mcg/kg/min. The effects of dopamine on heart rate appear to be dose dependent, with tachycardia contributing significantly to the rise in cardiac index at infusion rates greater than 7.5 mcg/kg/min. In adults with cardiac dysfunction, dopamine lowers systemic vascular resistance (SVR) in doses up to 10 mcg/kg/min and increases SVR with doses greater than or equal to 10 mcg/kg/min. Dopamine increases myocardial oxygen consumption, but it also increases oxygen delivery, leaving the coronary sinus and mixed venous oxygen saturation unchanged.
Clinical correlation of the impact of low-dose dopamine on urine output and creatinine clearance in pediatrics is not well supported. Although case studies support the use of low-dose dopamine to augment urine output, the literature to support its use is insuffient.
Management of the hypotensive premature infant represents a special circumstance in which dopamine infusions have been used. Systemic hypotension is associated with cerebral injury in very low-birth-weight infants. Although there is no significant difference between dopamine and dobutamine in the incidence of periventricular leukomalacia, grade III to IV intraventricular hemorrhage, or mortality, dopamine is more successful than dobutamine in short-term blood pressure elevation. Premature infants seem to require higher doses than children and adults for an equivalent inotropic effect; however, they demonstrate alpha effects at lower doses.
Common scenarios for administration of dopamine include (1) low cardiac output following cardiac surgery, (2) septic shock with low cardiac output and low SVR, and (3) premature infants with hypotension. (See also Chapter 16 on renal function.)
The major complications of dopamine therapy in children are extravasation and arrhythmia. Given the potential for tissue necrosis and gangrene, central administration is the preferred route of administration. Supraventricular tachydysrhythmias have been reported after dopamine infusion in infants and children. Risk factors include a preexisting supraventricular rhythm disturbance and high-dose dopamine (10 to 20 mcg/kg/min). Increased frequency of premature ventricular beats occurs at dopamine greater than 5 mcg/kg/min.
Dobutamine
Dobutamine is a synthetic catecholamine that improves the inotropic state of the heart with little effect upon chronotropy. Dobutamine acts primarily on beta 1 receptors, with some beta 2 and alpha effect. Unlike dopamine, dobutamine does not release norepinephrine. Dobutamine used clinically contains two enantiomeric forms. The (−) isomer is a potent alpha agonist, causing an increase in vascular resistance. However, the (+) isomer is a potent beta agonist as well as an alpha antagonist, which blocks the effects of the (−) isomer. Dobutamine is metabolized by glucuronidation via COMT.
Most studies indicate that plasma dobutamine concentrations increase proportionally with increasing infusion rate (i.e., linear kinetics). However, other studies showed that higher doses resulted in a smaller increase in concentration (e.g., nonlinear kinetics). Among children in the pediatric intensive care unit, the t 1/2 α of dobutamine was 1.65 ± 0.2 minutes, and the t 1/2 β was 25.8 ± 11.5 minutes. Half-lives did not correlate with age, weight, gender, disease state, or duration of dobutamine infusion. The half-lives were shorter when dopamine was coadministered. There seems to be a shift in the dose-response curve in younger (less than 1 year of age) children, leading to younger children needing a higher dose than adults to achieve the same pharmacologic effect.
The myocardial effects of dobutamine are not completely explained by its beta 1 -adrenergic receptor stimulation. Interestingly, there is an increase in cardiac index that results from an increase in stroke volume index without a significant increase in heart rate. At low infusion doses (less than 5 mcg/kg/min), children experience a decrease in pulmonary capillary wedge pressure and an increase of cardiac output and blood pressure without an increase in heart rate (see Table 19.1 ). At higher doses (greater than 5 mcg/kg/min), there is an associated increase in heart rate ( Fig. 19.4 ; see Table 19.1 ). SVR is either unchanged or decreased after dobutamine. There is no effect on pulmonary vascular resistance (PVR). The effect of dobutamine may be different following CPB in children. However, data are inconsistent because some studies have shown a more pronounced chronotropic effect without an impact on stroke volume and other studies have shown a significant increase in heart rate with an increase in cardiac index and SVR.

Dobutamine may be useful for patients admitted with decompensated systolic heart failure to improve inotropy and augment diuresis and heart failure symptoms or for patients with hemodynamically significant aortic or mitral regurgitation requiring afterload reduction and inotropy. Of note, adult data have shown increased in-hospital mortality, heart failure readmissions, and arrhythmias associated with the use of dobutamine compared to nesiritide for treatment of acute decompensated heart failure. Dobutamine causes fewer arrhythmias than epinephrine or isoproterenol.
Epinephrine
Epinephrine is a mixed beta 1 and beta 2 agonist at low doses and an alpha agonist at higher doses. Thus it functions as combined inotrope and chronotrope, and at higher doses as a vasoconstrictor.
The pharmacokinetics of epinephrine in critically ill children is variable but correlates with age and body weight. Endogenous production of epinephrine is based upon enzymatic maturation and negligible during epinephrine infusions given the near 50-fold increase in concentration while on an infusion. Epinephrine infusion rates designed to increase myocardial contractility (0.03 to 0.2 mcg/kg/min) yield plasma epinephrine levels of 670 to 9430 pg/mL. Plasma epinephrine concentrations vary linearly with epinephrine infusion rate, suggesting first-order kinetics. The half-life (t 1/2 ) of epinephrine is approximately 2 minutes. Epinephrine is degraded via the COMT and MAO systems.
Epinephrine is used frequently in the management of children with septic shock or low cardiac output syndrome (LCOS) after cardiac surgery when other inotropic agents have failed. Early administration of epinephrine was associated with increased survival when compared to dopamine in a single-center double-blind, prospective, randomized controlled trial of patients 1 month to 15 years of age with fluid-refractory septic shock, whereas dopamine was related to an increased risk of death and health care–associated infection.
Epinephrine infusion (3 to 30 mcg/min) improves oxygen delivery by increasing cardiac index without increasing SVR in adults with septic shock unresponsive to fluid resuscitation. After open-heart surgery, adult patients demonstrate a marked increase in cardiac output at infusion rates of 0.02 to 0.08 mcg/kg/min.
As epinephrine infusion rates increase, alpha 1 agonist effects predominate, and at the highest infusion rates SVR is greatly increased and cardiac index begins to fall. Newborns may be more susceptible than adults to myocardial injury, including sarcolemmal rupture and mitochondrial Ca 2+ granule deposition after prolonged high-dose epinephrine infusion.
The pulmonary vascular bed contains alpha and beta 2 receptors, so that pulmonary vasoconstriction (alpha stimulation) or vasodilation (beta 2 stimulation) can be expected depending on a variety of circumstances. At low and medium doses (<0.8 mcg/kg/min), epinephrine decreases PVR and increases pulmonary blood flow. Ventilation/perfusion mismatch may result. Higher doses appear to raise PVR if the preinfusion PVR was normal. Conversely, if the preinfusion PVR was elevated by either hypoxia or sepsis, even high-dose epinephrine administration (1 to 3.5 mcg/kg/min) may yield predominantly beta 2 -adrenergic stimulation and pulmonary vasodilation.
The effects of epinephrine infusion on regional blood flow have been evaluated primarily in animal studies showing renal vascular resistance increases in a dose-dependent manner with epinephrine infusion in adult sheep. Similarly, newborn piglets demonstrate decreased superior mesenteric artery, hepatic, and renal blood flow with epinephrine (less than 3 mcg/kg/min). Following cardiac surgery in adults, epinephrine administration (0.04 mcg/kg/min) reduces the ratio of renal blood flow to cardiac index, whereas this ratio is not changed with dobutamine (2 to 8 mcg/kg/min) and is improved with dopamine (4 mcg/kg/min).
Epinephrine is still considered the drug of choice in cardiopulmonary resuscitation (CPR) (see Chapter 31 ). In a witnessed pediatric cardiac arrest the standard initial dose of epinephrine is 10 mcg/kg, and high-dose epinephrine (100 mcg/kg) has been controversial in the past, including a randomized controlled trial indicating that high-dose epinephrine may actually worsen outcomes after cardiac arrest in children. Bolus administration of epinephrine to a compromised myocardium may result in ventricular fibrillation.
Epinephrine can be administered down the endotracheal tube at a dose of 100 mcg/kg, 10 times the recommended intravenous (IV) dose, resulting in rapid absorption and an increase in systolic blood pressure.
Patients with depressed ventricular function, low cardiac output, and systemic hypotension can benefit from epinephrine infusion. It should be avoided in patients at high risk for ventricular arrhythmia.
Hypokalemia and hyperglycemia represent the most common metabolic side effects during epinephrine administration. Hypokalemia results from K + uptake into skeletal muscle cells after beta 2 -receptor stimulation. Hyperglycemia results from suppressed insulin release as well as increased glycogenolysis and gluconeogenesis.
Epinephrine may cause extravasation and skin necrosis when administered via non–central venous access. For this reason, epinephrine infusions should be administered through a central venous access device whenever possible.
The most serious side effect of epinephrine is ventricular arrhythmia. Myocarditis, hypokalemia, and hypercapnia, particularly in the presence of inhaled anesthetics such as halothane, predispose patients to ventricular arrhythmia during epinephrine administration.
Norepinephrine
Norepinephrine is an endogenous catecholamine that is the neurotransmitter at sympathetic postganglionic fibers (see Fig. 19.1 ). It has potent beta 1 – and alpha-stimulating effects. In contrast to epinephrine, norepinephrine has only minor effects on beta 2 receptors.
Variability in norepinephrine pharmacokinetics is related to body weight, age, and severity of illness. Norepinephrine has a short half-life. After secretion of norepinephrine by the nerve endings, most of it is reabsorbed (where MAO can degrade it); the remainder diffuses into the bloodstream, where it remains active for at most minutes. Like epinephrine, norepinephrine is degraded via the COMT and MAO systems
The clinical effects of norepinephrine administration are mainly increased cardiac index and increased vascular (systemic and pulmonary) resistance. Several adult studies have suggested that norepinephrine is useful in increasing SVR in patients with hyperdynamic or vasodilatory septic shock that is not responsive to dopamine or epinephrine. Additionally, it can augment coronary blood flow by increasing systemic diastolic pressure, at the expense of increasing afterload.
Given the significant vasoconstrictive properties of norepinephrine, the risk of extravasation and skin necrosis upon administration is quite high, and therefore it should be administered via central venous access.
Isoproterenol
Isoproterenol is a nonspecific beta agonist with no alpha-adrenergic activity and therefore increases inotropy, chronotropy, and systemic and pulmonary vasodilation.
Isoproterenol has a short half-life of 2 to 5 minutes, requiring a continuous infusion. Clearance is mostly in the urine as sulfate conjugates. Postoperative cardiac patients have lower clearance rates and require significantly lower infusion rates than patients with reactive airway disease to achieve clinical effect, potentially due to differences in metabolism, distribution related to plasma protein binding, and cardiac output and related perfusion of the liver and kidneys. The major degradative pathway for isoproterenol is via COMT.
In vitro isoproterenol has greater positive inotropic effects in newborns than in adults. Systolic blood pressure is increased, and diastolic and mean blood pressures are decreased because of systemic vasodilation.
Isoproterenol may increase ventricular escape rate in cases of complete heart block. It is used to maintain heart rate, decrease afterload, and decrease right ventricular pressures immediately following heart transplantation. Isoproterenol may be administered through peripheral IV access.
Arrhythmia, both atrial and ventricular, can occur with isoproterenol. Isoproterenol should be avoided in patients with hypertrophic cardiomyopathy, fixed outflow tract obstruction, or compromised coronary blood flow because it can increase myocardial oxygen consumption and decrease coronary perfusion pressure. When it is used to stabilize complete heart block or effects of beta-blockade, hypotension can occur if the isoproterenol fails to effectively increase the heart rate. Due to potential risk of myocardial ischemia, isoproterenol has been replaced by the more beta 2 -specific terbutaline in treatment of the child with status asthmaticus.
Milrinone
Milrinone is a bipyridine derivative that inhibits cyclic nucleotide phosphodiesterase (III) resulting in increased cAMP. Increased cAMP causes an increase in intracellular ionic calcium concentration and therefore improved myocardial contractility by increasing calcium transit and myofilament binding. In the systemic vasculature, increased cAMP results in relaxation, decreasing afterload.
Phosphodiesterase III inhibitors may have antiinflammatory effects in septic shock. In vitro cardiac myocytes exposed to tumor necrosis factor-α (TNF-α) have a reduced contractile response to epinephrine compared with controls, whereas myocytes exposed to TNF-α have an augmented contractile response to phosphodiesterase III inhibitor compared with controls.
Milrinone is primarily excreted by the kidneys as an unchanged drug (83%) and a glucuronide metabolite (12%) and must be used with caution in patients with renal impairment.
For infants and children the typical loading dose for milrinone is 50 mcg/kg with an infusion at 0.2 to 1 mcg/kg/min.
Milrinone pharmacokinetics in pediatrics has been studied primarily following cardiac surgery. Milrinone does not bind to the bypass circuitry. Milrinone steady state volume of distribution is larger in infants (0.9 L/kg) compared with children (0.7 L/kg) and three times that of adults (0.3 L/kg). The elimination clearances rates for milrinone following CPB are lower for infants compared with children but twofold to threefold greater than milrinone clearance in adults. The half-life (t 1/2 ) of milrinone is shortest in adults at 1.7 hours compared with children and infants (1.9 hours and 3 hours).
In adults with significant chronic congestive failure, phosphodiesterase inhibitors improve cardiac index, reduce left ventricular filling pressures, and improve the speed of contraction without significant side effects such as tachycardia. After cardiac surgery in adults and neonates, cardiac index increases and heart rate increases, whereas systemic and pulmonary vascular resistance decrease with milrinone administration ( Figs. 19.5 and 19.6 ). These findings were most pronounced in those individuals with heart failure.


Administration of phosphodiesterase inhibitors to children with left-to-right intracardiac shunts resulted in a more dramatic decrease in PVR in those patients with baseline elevation of their pulmonary artery pressures and PVR compared with those with normal pulmonary artery pressures and PVR.
Barton and colleagues administered milrinone to children with nonhyperdynamic septic shock and demonstrated that milrinone significantly increased cardiac index, increased stroke volume index, and increased oxygen delivery while decreasing systemic and pulmonary vascular resistance and not affecting heart rate.
Milrinone is used following cardiac surgery to augment inotropy and decrease SVR during periods of low cardiac output ( Table 19.2 ). Hoffman and colleagues demonstrated in a double-blind, placebo-controlled multicenter trial in infants and children following corrective heart surgery (n = 227) that milrinone (0.75 mcg/kg/min) significantly decreased the incidence of LCOS compared with placebo ( Fig. 19.7 ). A meta-analysis confirmed the efficacy of prophylactic milrinone in reducing the incidence of LCOS but concluded there is insufficient evidence that prophylactic milrinone reduces mortality. Milrinone is also an excellent agent for dilated cardiomyopathy due to myocarditis, graft rejection, and sepsis-associated cardiac dysfunction and a potential therapy as a bridge to transplantation in patients with advanced heart failure. Additionally, milrinone can be used as adjunct therapy in managing pulmonary hypertension. Milrinone can improve cardiac index and oxygen delivery in children with nonhyperdynamic septic shock. Preliminary data suggest that some phosphodiesterase inhibitors reduce systemic inflammatory response by reducing tumor necrosis factor and interleukin-1.
Drug | Dose | Clinical Effects | Usual Titration a | Cautions/Comments |
---|---|---|---|---|
Esmolol | Load: 100-500 mcg/kg infused over 1 min |
| 50-100 mcg/kg/min every ≥10 min until desired effect is reached
|
|
Usual starting: 50-100 mcg/kg/min Usual range: 50-250 mcg/kg/min | ||||
MAX: 1000 mcg/kg/min | ||||
Milrinone | Load: 50-100 mcg/kg |
| 0.125-0.25 mcg/kg/min every ≥30 min until desired effect is reached |
|
Usual starting: 0.5-1 mcg/kg/min Usual range: 0.25-1 mcg/kg/min | ||||
MAX: 1.5 mcg/kg/min | ||||
Nitroglycerin | Usual starting: 0.25-0.5 mcg/kg/min Usual range: 0.25-3 mcg/kg/min |
| 0.5-1 mcg/kg/min every ≥3 min until desired effect is reached |
|
MAX: 5 mcg/kg/min
MAX adult dose : 400 mcg/min | ||||
Nitroprusside | Usual starting: 0.3-0.5 mcg/kg/min Usual range: 1-3 mcg/kg/min |
| 0.5 mcg/kg/min every ≥5 min until desired effect is reached |
|
MAX: 10 mcg/kg/min
MAX adult dose : 400 mcg/min | ||||
Vasopressin (pressor effect) | Usual starting: 0.05 mU/kg/min Usual range: 0.05-2 mU/kg/min |
| 0.05-0.5 mU/kg/min every ≥30 min until desired effect is reached |
|
a Titrations may vary based upon clinical indication and patient status.

Hypotension is the most frequent cardiovascular side effect due to the vasodilatory properties noted previously, which may be prevented by alleviating the loading dose, administering a lower dose or administering the loading dose more slowly (over 10 to 20 minutes), and/or by concomitant volume expansion. Milrinone should be avoided in patients with severe left or right heart outflow tract obstruction because of the risk that fixed outflow tract obstruction in the presence of vasodilation may compromise coronary perfusion.
Thrombocytopenia has been reported with milrinone in patients following cardiac surgery, but there was no nonsurgical control group. Other studies found no effect of milrinone on platelet count or function.
Calcium
In the past, calcium was routinely administered in situations where improved cardiac function was needed. In newborn lambs with single-ventricle physiology, Reddy and colleagues demonstrated that administration of calcium (10 mg/kg of calcium chloride) significantly increased systolic blood pressure and decreased pulmonary-to-systemic blood flow ratio (Q p :Q s ) prebypass ( Table 19.3 ). The maximal inotropic effect of calcium in the newborn was significantly greater than in adults, perhaps because of a lower intracellular calcium concentration. Calcium is of particular importance in newborns with congenital heart disease (CHD), particularly those with conotruncal abnormalities, for which there is an increased prevalence of 22q11 deletion (DiGeorge syndrome) and associated hypoparathyroidism.
PAP (mm Hg) | SAP (mm Hg) | Q p (mL/min/kg body weight) | Q s (mL/min/kg body weight) | Q p :Q s | PVR (mm Hg/min/kg) | SVR (mm Hg/ min/kg) | |
---|---|---|---|---|---|---|---|
Before Cardiopulmonary Bypass | |||||||
Precalcium | 31.7 ± 5.4 | 55.5 ± 11.6 | 249 ± 30 | 130 ± 39 | 2.09 ± 0.80 | 0.101 ± 0.031 | 0.41 ± 0.15 |
Postcalcium | 32.0 ± 5.4 | 59.0 ± 12.5 | 268 ± 26 b | 150 ± 41 c | 1.90 ± 0.58 d | 0.100 ± 0.025 | 0.38 ± 0.14 d |
After Cardiopulmonary Bypass | |||||||
Precalcium | 28.6 ± 4.4 | 51.7 ± 8.8 | 238 ± 55 | 149 ± 49 | 1.71 ± 0.55 | 0.101 ± 0.027 | 0.34 ± 0.14 |
Postcalcium | 28.9 ± 4.0 | 58.0 ± 7.8 d | 268 ± 62 a | 174 ± 50 c | 1.60 ± 0.44 | 0.090 ± 0.025 c | 0.32 ± 0.11 |
a Neonatal lambs with single-ventricle physiology created in utero (Damus-Kaye-Stansel, main pulmonary artery ligation, and placement of 5-mm aortopulmonary shunt) had direct measurement of systemic and pulmonary blood flow both before and after cardiopulmonary bypass 48 to 72 hours postnatally. Administration of calcium (calcium chloride 10 mg/kg) increased systemic arterial pressure both prebypass and postbypass and decreased Q p :Q s prebypass.
c P < .0001, relative to preadministration values.
Studies in adults have suggested that calcium is ineffective in the face of refractory asystole, yet of potential benefit in a subset of patients with refractory electromechanical dissociation. Current CPR guidelines discourage use of calcium for treatment of asystole and recommend its use in cases of hyperkalemia, hypermagnesemia, and calcium channel blocker overdose.
Significant hypocalcemia is very common in critically ill children, is associated with poor outcomes, and may be due to relative or absolute hypoparathyroidism. Modestly low calcium levels are not related to impaired cardiac function, but infusions of calcium in this setting can improve mean arterial pressures. In adults following CPB, calcium increases blood pressure without improving cardiac index. However, in infants and children, maintaining ionized calcium levels is important for maintaining blood pressure and cardiac output, perhaps due to relatively diminished intracellular calcium stores or perhaps due to the relative increased volume of transfused citrated blood products. Calcium chloride infusions in pediatric patients with LCOS have been shown in a small retrospective study to improve hemodynamics and end-organ perfusion without an increase in heart rate and without relation to low baseline ionized calcium.
Thyroid Hormone
Thyroid hormone directly affects inotropy and peripheral vascular resistance. Thyroxine (T 4 ) is synthesized by the thyroid gland and converted by deiodinase enzymes to the active compound triiodothyronine (T 3 ). T 3 increases synthesis of the fast alpha-myosin heavy chain, increases sarcoplasmic reticulum Ca 2+ adenosine triphosphatase, and increases intracellular cAMP, resulting in increased inotropy and decreased peripheral vascular resistance.
Thyroid hormone abnormalities, including decreased T 3 , normal or decreased T 4 and thyrotropin, and increased reverse T 3 , are well documented in children following cardiac surgery and in adults following cardiac bypass, post myocardial infarction, and with severe congestive heart failure. In adults with congestive heart failure, a low T3:reverse T 3 index was associated with lower cardiac index, elevated filling pressures, and poorer outcome. Euthyroid sick syndrome is a constellation of abnormal thyroid function test results in a clinically euthyroid patient who is suffering from critical illness that appears to involve decreased peripheral conversion of T 4 to T 3 , decreased clearance of reverse T 3 generated from T 4 , and decreased binding of thyroid hormones to thyroid-binding globulin.
Administration of T 3 to adults following coronary artery bypass surgery and heart failure improved hemodynamic parameters increasing cardiac output and decreased SVR ( Fig. 19.8 ), whereas Bennett-Guerrero and colleagues found no significant change in cardiac index or SVR. Currently T 3 seems more beneficial in the setting of congestive heart failure or dilated cardiomyopathy. Bettendorf and colleagues found that T 3 administration to infants increased cardiac index during the first 24 hours after cardiac surgery, particularly for those with prolonged CPB (>1.8 hours), compared to the placebo group, and by 72 hours both groups had similar cardiac indices and T 3 levels. Chowdhury et al. studied T 3 infusions post CPB with T 3 level of less than 40 ng/dL (or <60 ng/dL for newborns) and found that the T 3 infusion group had significantly lower inotropic requirements. The Transfusion Requirements in Critical Care (TRICC) study was a large multicenter, double-blind, randomized, placebo-controlled trial in pediatric cardiac surgery patients less than 2 years of age that evaluated the use of IV T 3 post CPB. Results of the study were significant in patients less than 5 months of age, resulting in a shortened time to extubation correlating to reduction in inotrope use and improved cardiac function.

Digoxin
Digoxin is a cardiac glycoside that inhibits Na + -K + ATPase in the cardiomyocyte, resulting in increased intracellular Na + concentration, thereby increasing intracellular Ca 2+ through a Na + -Ca 2+ exchange mechanism. Contractile force and velocity depend on the concentration of and sensitivity to Ca 2+ in the contractile apparatus. The ability of digoxin to drive the Na + -Ca 2+ exchanger may be limited in the immature heart.
Digoxin undergoes metabolism in the liver and limited enterohepatic circulation. In addition, bacteria in the large intestine may metabolize the drug. Unmetabolized digoxin and metabolites are then excreted in urine and feces.
Absorption of oral digoxin from the gastrointestinal tract is variable from one individual to another and dependent upon the formulation. The onset of action after an oral dose occurs within 30 to 120 minutes and peaks at approximately 6 to 8 hours. The effects of IV digoxin begin after 5 to 30 minutes. The elimination half-life in adult patients with normal renal function is between 36 and 48 hours and prolonged in renal dysfunction. In preterm neonates and full-term neonates the half-life is 60 to 170 hours and 35 to 45 hours, respectively. In toddlers and younger children the half life is 18 to 35 hours ( Table 19.4 ).
Digoxin Loading/Digitalizing Dose Table (mcg/kg per dose) a-h | ||||||
ORAL | INTRAVENOUS | |||||
First Dose | Second Dose | Third Dose | First t Dose | Second Dose | Third Dose | |
Preterm neonate | 10-15 | 5-7.5 | 5-7.5 | 7.5-12.5 | 3.75-6.25 | 3.75-6.25 |
Full-term neonate | 12.5-17.5 | 6.25-8.75 | 6.25-8.75 | 10-15 | 5-7.5 | 5-7.5 |
1 mo-2 y | 17.5-30 | 8.75-15 | 8.75-15 | 15-25 | 7.5-12.5 | 7.5-12.5 |
2-5 y | 15-20 | 7.5-10 | 7.5-10 | 12.5-17.5 | 6.25-8.75 | 6.25-8.75 |
5-10 y i | 10-17.5 | 5-8.75 | 5-8.75 | 7.5-15 | 3.75-7.5 | 3.75-7.5 |
>10 y i | 5-7.5 | 2.5-3.75 | 2.5-3.75 | 4-6 | 2-3 | 2-3 |
ADULT maximum dose (Do NOT Exceed) | 500 mcg (FLAT dose) | 250 mcg (FLAT dose) | 250 mcg (FLAT dose) | 500 mcg (FLAT dose) | 250 mcg (FLAT dose) | 250 mcg (FLAT dose) |
Digoxin Maintenance Dose Table (mcg/kg per dose) | ||
Oral | Intravenous | |
Preterm neonate | 2.5-3.75 mcg/kg/dose q12h | 2-3 mcg/kg/dose q12h |
Full-term neonate | 3-5 mcg/kg/dose q12h | 2.5-4 mcg/kg/dose q12h |
1 mo-2 y | 5-7.5 mcg/kg/dose q12h | 3.75-6 mcg/kg/dose q12h |
2-5 y | 3.75-5 mcg/kg/dose q12h | 3-4.5 mcg/kg/dose q12h |
5-10 y i | 2.5-5 mcg/kg/dose q12h | 2-4 mcg/kg/dose q12h |
>10 y i | 2.5-5 mcg/kg/dose once daily | 2-3 mcg/kg/dose once daily |
Usual ADULT dose (Do NOT Exceed) | 125-375 mcg once daily (FLAT dose) | 125-375 mcg once daily (FLAT dose) |
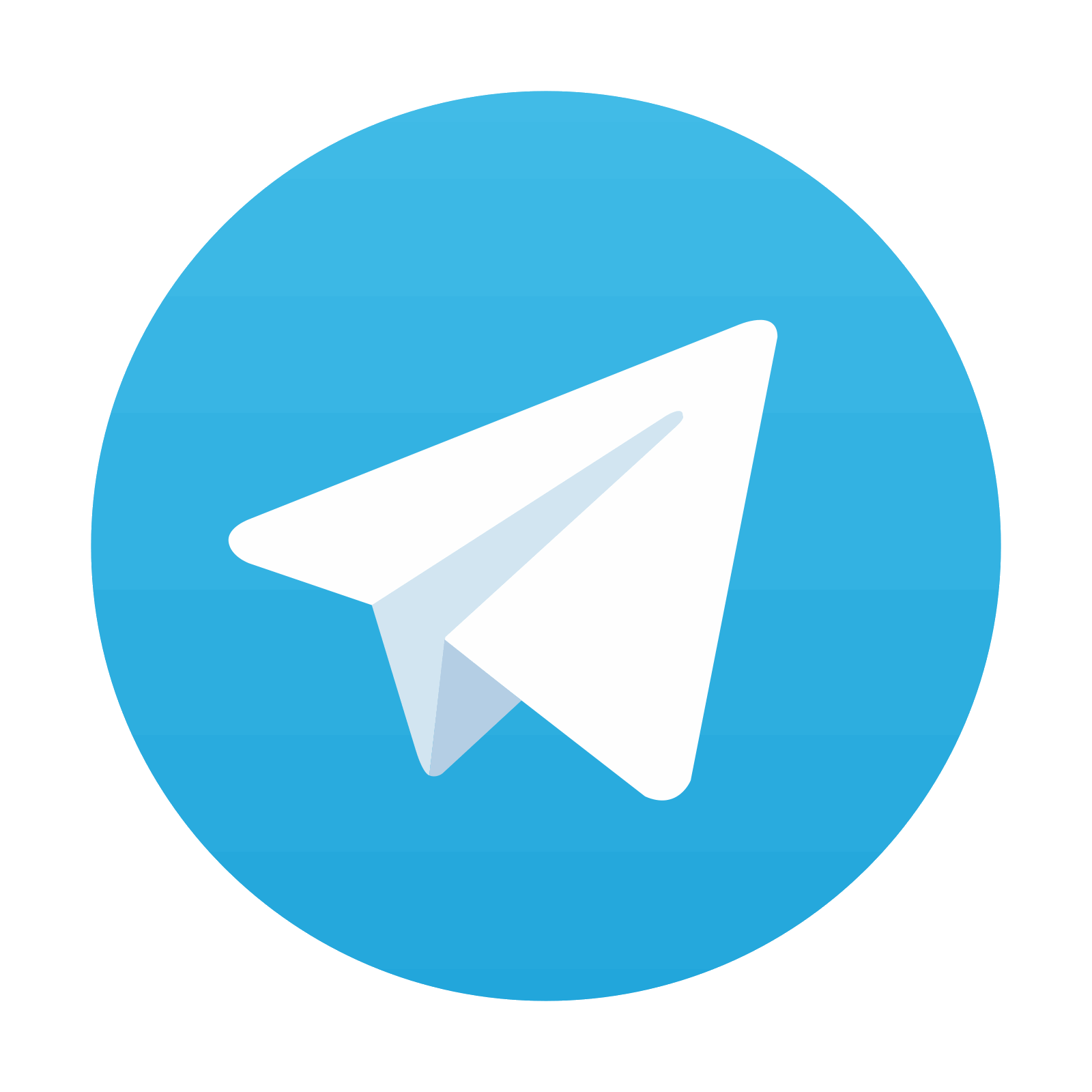
Stay updated, free articles. Join our Telegram channel
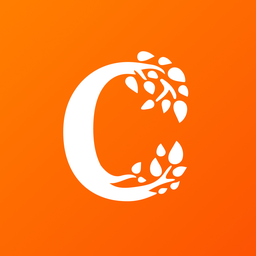
Full access? Get Clinical Tree
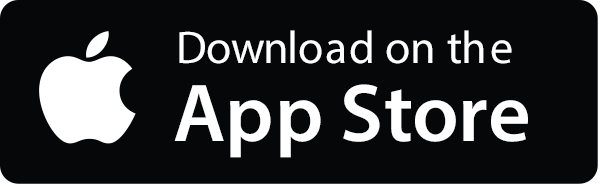
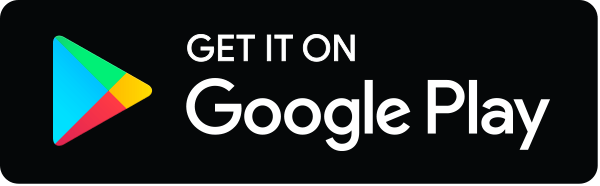
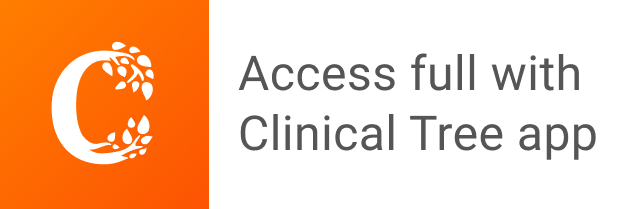