Nerve
Radiotracer
Description
Sympathetic
Presynaptic
11C-hydroxyephedrine (HED)
Most widely used PET-based radiotracer for cardiac sympathetic innervation
NE analogue, accumulates through NET and transported to storage vesicles by VMAT2
Not metabolized by MAO or COMT, excellent-quality images for quantitation
Short half-life (20 min) isotope, requires on-site cyclotron
11C-epinephrine
Radiolabeled neurotransmitter that behaves similarly to NE
Uptake, storage, and degradation mimic those of NE
Cleared slowly because of its vesicular storage
11C-phenylephrine
Radiolabeled synthetic catecholamine with limited human studies
Lower affinity for NET than HED
Demonstrates vesicular leakage and enzymatic degradation by MAO, contributing to significant myocardial washout
18F-fluorodopamine
Longer half-life (2 h) isotope, permitting regional tracer synthesis
Physiologic tracer forming labeled NE
Difficult to manufacture and requires complex modeling
18F-LMI 1195
Accumulates through NET uptake
Well-defined cardiac uptake, low washout, and low background activity
Postsynaptic
11C-CGP 12177
Nonselective, hydrophilic β-receptor antagonist
High specificity with low background activity
Similar affinity for β1– and β2-receptors and lower affinity for β3-receptor
Difficult production of 11C-phosgene, which is a required intermediate
Parasympathetic
Presynaptic
Vesamicol derivatives
Selective inhibitors of vesicular acetylcholine transporter
Low tracer retention, high nonspecific binding, and rapid washout
Postsynaptic
11C-MQNB
M2-receptor antagonist
High receptor specificity in human studies
PET Radiotracers for Evaluation of Cardiac Autonomic Innervation
PET radiotracers used for imaging cardiac innervation may be classified based on their principal targets; that is, sympathetic versus parasympathetic nerve terminals and presynaptic versus postsynaptic components. The different radiotracers are summarized in Table 5.1.
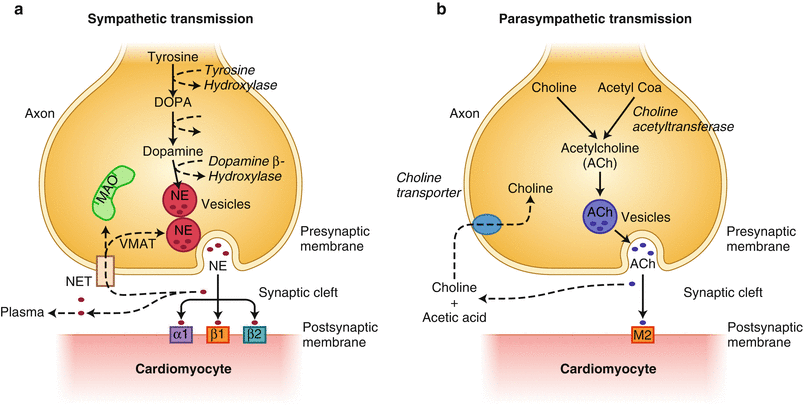
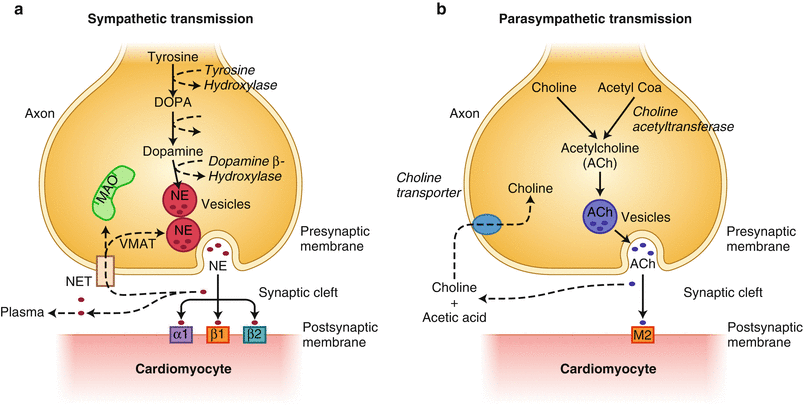
Figure 5-1.
Schematic diagram of cardiac sympathetic and parasympathetic innervation. The cardiac network of neural innervation is composed primarily of two divisions, sympathetic (a) and parasympathetic (b). The sympathetic nervous system generally promotes positive inotropic and chronotropic responses through its major neurotransmitter, norepinephrine (NE), exerting its major cellular effects through binding to adrenergic receptors (predominantly β-receptors, β1/β2-receptors expressed in ~7:3 ratio). The parasympathetic nervous system generally suppresses cardiac inotropic and chronotropic responses through the release of its primary neurotransmitter, acetylcholine (ACh), binding to muscarinic receptors (M). (a) Within the presynaptic sympathetic nerve terminal, NE is synthesized through a series of enzymatic steps starting from the conversion of tyrosine to eventual formation of NE. Following presynaptic sympathetic nerve activation, docking of vesicles to the axonal membrane releases stored NE into the synaptic cleft. NE is actively recaptured from the synaptic cleft by NE transporter (NET) by the so-called uptake-1 mechanism. This saturable and ATP-dependent mechanism allows NE recycling and repackaging into vesicles. (b) Parasympathetic nerves are less abundant overall than sympathetic nerves, and primarily affect the atria rather than the ventricles. Stimulated parasympathetic postganglionic neurons release ACh, which binds and activates primarily M2 muscarinic receptors expressed in cardiomyocytes. ACh is enzymatically degraded by acetylcholinesterase. DOPA dihydroxyphenylalanine, MAO monoamine oxidase, VMAT vesicular monoamine transporter.
Principles of PET Image Acquisition, Quantitation, and Interpretation
Cardiac PET imaging typically requires acquisition of a series of sequential dynamic images designed to provide information regarding tracer uptake, redistribution, decay, and dispersion. The sequence of images is designed to incorporate a minimum number of time frames that would allow analysis of tracer kinetics while ensuring adequate temporal resolution. Carbon-11 (11C)-hydroxyephedrine (HED)-based imaging of sympathetic innervation is the most widely studied and therefore is used in the following section as a reference for discussion. Each of the following principles is applicable to other cardiac sympathetic markers, as well. Figure 5.2 describes the framework with which PET tracer kinetics may be used to probe physiologic systems.
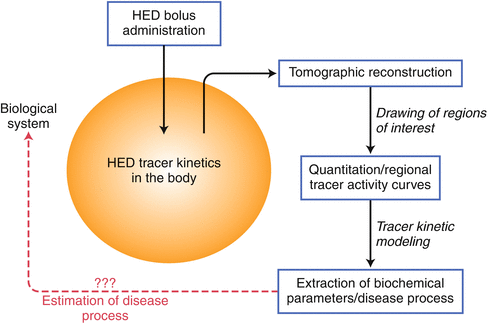
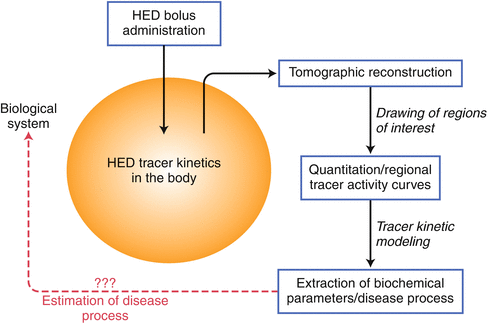
Figure 5-2.
Framework for using sympathetic PET radiotracers for analyzing a biologic system. The initial step requires identification of an appropriate PET radiotracer to address a clinical question. The use of 11C-hydroxyephedrine (HED) is well described and is the most frequently used radiotracer for probing sympathetic innervation. Following bolus intravenous administration of HED, its kinetics within the body reflects the summation of several physiologic processes within a specific biologic system (i.e., tracer distribution, retention, and washout). Its positron emission properties allow three-dimensional tomographic reconstruction and quantitation of dynamic time–activity curves. Based on the generated tracer activity, kinetic modeling may be applied to allow indirect estimation of different biochemical properties, such as tracer diffusion from blood to the interstitial layer or the rate of active uptake mechanism, among others. These estimated parameters derived from kinetic modeling may help define pathologic processes occurring within the biologic system.
Quantitation of Myocardial HED Uptake
Myocardial HED Radiotracer Time–Activity Curve
Image analysis may be approached several ways, from semiquantitative to the more sophisticated biochemical kinetic modeling. From the serial HED PET images, absolute myocardial tracer uptake may be obtained and analyzed. Following intravenous administration, HED rapidly accumulates in the myocardium and is cleared from the blood pool. Because of HED’s rapid uptake and blood pool washout, emission data reflecting relative myocardial uptake of HED may be acquired within 40–60 min from the time of intravenous bolus administration. A dynamic sequence of image frames is obtained during the acquisition period (Fig. 5.3). Frequently, a shorter time interval between frames is ideal during the early time frame to capture the fast tracer uptake adequately. For the latter time frame, a longer time interval between images is typical to obtain sufficient photons as the tracer redistributes and decays. Raw data are obtained as a series of transaxial planes that may then be reconstructed into standard conventional tomographic views; that is, short axis, horizontal long, and vertical long axis views. Myocardial regions of interest may be identified and activity sampled over the entire indicated time frames, generating time–activity curves. Myocardial tracer activity is quantified as counts/pixel, which may be converted to megabecquerels per tissue volume from the machine calibration factor. To improve imaging accuracy, several corrections may be applied, including tissue attenuation correction using transmission scans of PET-emitting isotope source, partial volume correction to minimize systematic underestimation of small objects due to low camera spatial resolution [1, 2], and spillover corrections to account for heterogeneous uptake in adjacent structures.
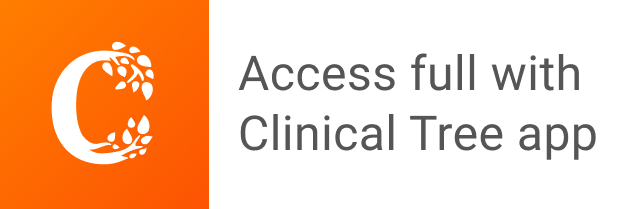