Clinical Cyanosis
Detection of Cyanosis
Cyanosis is a bluish discoloration of the skin and mucous membranes resulting from an increased concentration of reduced hemoglobin to about 5 g/100 mL in the cutaneous veins. This level of reduced hemoglobin in the cutaneous vein may result from either desaturation of arterial blood or increased extraction of oxygen by peripheral tissue in the presence of normal arterial saturation (e.g., circulatory shock, hypovolemia, vasoconstriction from cold). Cyanosis associated with desaturation of arterial blood is called central cyanosis; cyanosis with normal arterial oxygen saturation is called peripheral cyanosis.
Cyanosis is more difficult to detect in children with dark pigmentation. Although cyanosis may be detected on many parts of the body, including the lips, fingernails, oral mucous membranes, and conjunctivae, the tip of the tongue is a good place to look for cyanosis; the color of the tongue is not affected by race or ethnic background, and the circulation is not sluggish in the tongue. In a newborn, acrocyanosis may cause confusion. In addition, some newborns are polycythemic, which may contribute to the appearance of cyanosis without arterial desaturation (see later discussion). In older infants and children, chronic subclinical cyanosis produces clubbing.
When in doubt, arterial oxygen saturation should be obtained by a pulse oximeter or arterial Po 2 by blood gas determination. Normal Po 2 in a 1-day-old infant may be as low as 60 mm Hg. An arterial oxygen saturation of 90% or above does not completely rule out a cyanotic heart defect in a newborn infant. An arterial oxygen saturation of 90% can be seen with a Po 2 of 45 to 50 mm Hg in newborns because of the normally leftward oxygen hemoglobin dissociation curve (see later section). In older children and adults, a Po 2 of 60 to 65 mm Hg is needed to have 90% oxygen saturation.
Influence of Hemoglobin Level on Cyanosis
The level of hemoglobin greatly influences the occurrence of cyanosis. This effect is graphically illustrated in Figure 11-1 . As stated earlier, about 5 g/100 mL of reduced hemoglobin in cutaneous veins is required for the appearance of cyanosis. Normally, about 2 g/100 mL of reduced hemoglobin is present in the venules so that an additional 3 g/100 mL of reduced hemoglobin in arterial blood produces clinical cyanosis. For a normal person with hemoglobin of 15 g/100 mL, 3 g of reduced hemoglobin results from 20% desaturation (because 3 is 20% of 15). Thus, cyanosis appears when the oxygen saturation is reduced to about 80%. Cyanosis is recognized at a higher level of oxygen saturation in patients with polycythemia and at a lower level of oxygen saturation in patients with anemia (see Fig. 11-1 ). For example, in a person with polycythemia with hemoglobin of 20 g/100 mL, 3 g of reduced hemoglobin results from only 15% desaturation (or at 85% arterial saturation). On the other hand, in a patient with a marked anemia (e.g., hemoglobin of 6 g/100 mL), cyanosis does not appear until arterial oxygen saturation is reduced to 50% (3 g of reduced hemoglobin results from 50% desaturation).

Causes of Cyanosis
Cyanosis may result from a number of causes ( Box 11-1 ). Central cyanosis (with reduced arterial oxygen saturation) may be caused by cyanotic congenital heart defects, lung disease, or central nervous system (CNS) depression. Cyanosis of cardiac origin must be diagnosed early for proper management, but the detection of mild cyanosis is not always easy.
Reduced arterial oxygen saturation (i.e., central cyanosis)
Inadequate Alveolar Ventilation
Central nervous system depression
Inadequate ventilatory drive (e.g., obesity, Pickwickian syndrome)
Obstruction of the airway, congenital or acquired
Structural changes in the lungs or ventilation–perfusion mismatch (e.g., pneumonia, cystic fibrosis, hyaline membrane disease, pulmonary edema, congestive heart failure)
Weakness of the respiratory muscles
Desaturated Blood Bypassing Effective Alveolar Units
Intracardiac right-to-left shunt (i.e., cyanotic congenital heart defect)
Intrapulmonary shunt (e.g., pulmonary arterioventricular fistula, chronic hepatic disease resulting in multiple microvascular fistulas in the lungs)
Pulmonary hypertension with the resulting right-to-left shunt at the atrial, ventricular, or ductal levels (e.g., Eisenmenger’s syndrome, persistent pulmonary hypertension of the newborn)
Increased deoxygenation in the capillaries (i.e., peripheral cyanosis)
Circulatory shock
Congestive heart failure
Acrocyanosis of newborns
Methemoglobinemia
Ingestion of toxic substances (well water, aniline dye)
Congenital methemoglobinemia
Rarely, cyanosis is caused by methemoglobinemia. Methemoglobinemia may occur as a hereditary disorder or be caused by toxic substances. Toxic methemoglobinemia (such as that seen with ingestion of water high in nitrate or exposure to aniline teething gels) is more common than hereditary methemoglobinemia (absence of reductive pathways, or NADH-cytochrome b5 reductase deficiency). When methemoglobin (MHg) levels are greater than 15% of normal hemoglobin, cyanosis is visible, and a level of 70% MHg is lethal. Compensatory polycythemia may occur in this condition. In methemoglobinemia, color of the blood may remain brown even after a full oxygenation or a long exposure to room air.
Acrocyanosis, a bluish color of the fingers seen in neonates and infants, is a form of peripheral cyanosis and reflects sluggish blood flow in the fingers. It has no clinical significance unless associated with circulatory shock. Circumoral cyanosis refers to a bluish skin color around the mouth. This is a form of peripheral cyanosis seen in a healthy child with fair skin because of a sluggish capillary blood flow in association with vasoconstriction. Isolated circumoral cyanosis is of no concern unless it occurs as a result of a low cardiac output.
Cyanosis of Cardiac Versus Pulmonary Origin
Differentiation of cardiac cyanosis from cyanosis caused by pulmonary diseases is crucially important for proper management of cyanotic infants. The hyperoxitest helps differentiate cyanosis caused by cardiac disease from that caused by pulmonary disease. In the hyperoxitest, one tests the response of arterial Po 2 to 100% oxygen inhalation. With pulmonary disease, arterial Po 2 usually rises to a level greater than 100 mm Hg. When there is a significant intracardiac right-to-left shunt, the arterial Po 2 does not exceed 100 mm Hg, and the rise is usually not more than 10 to 30 mm Hg, although some exceptions exist. See Chapter 14 for exceptions and further discussion.
Figure 11-2 explains why breathing 100% oxygen does not significantly increase Po 2 in the presence of a right-to-left intracardiac shunt. Figure 11-2 , A , is a schematic illustration of the effect of a right-to-left shunt on the Po 2 while breathing in room air. Assuming a cardiac output of 2 L/min, 1 L of venous blood is distributed to ventilated alveoli, and 1 L is shunted right to left through a cardiac defect. Mixing 1 L of venous blood with an oxygen content of 19.4 mL/100 mL (Po 2 of 30 mm Hg) with 1 L of pulmonary venous blood containing 26.3 mL/100 mL (Po 2 of 100 mm Hg) results in an oxygen content of 22.8 mL/100 mL. The corresponding Po 2 from the dissociation curve is 41 mm Hg. Therefore, mixing 1 L of blood with a Po 2 of 100 mm Hg with 1 L of blood with a Po 2 of 30 mm Hg results in a Po 2 of 41 mm Hg (see Fig. 11-2 , A ), not an arithmetic average of 65 mm Hg. With the patient breathing 100% oxygen (see Fig. 11-2 , B ), the alveolar Po 2 becomes 600 mm Hg (with a corresponding oxygen content of 28.6 mL/100 mL, assuming a hemoglobin level of 20 g/100 mL; this figure is derived from 26.8 mg/100 mL of oxygen bound to hemoglobin plus 1.8 mL of oxygen dissolved in plasma [0.003 × 600]). When 1 L of blood with a Po 2 of 600 mm Hg (oxygen content 28.6 mg/100 mL) is mixed with l L of venous blood with a Po 2 of 30 mm Hg (oxygen content of 19.4 mL/100 mL), the resulting oxygen content is 24 mg/100 mL ([28.6 + 19.4]/2), with a corresponding Po 2 of 46 mm Hg (see Fig. 11-2 , B ). Thus, breathing 100% oxygen does not significantly alter the Po 2 (an increase from 41 to 46 mm Hg) even though the alveolar Po 2 increases from 100 to 600 mm Hg.

Hemoglobin Dissociation Curve
A full understanding of the hyperoxitest and the unique behavior of fetal hemoglobin requires the knowledge of the hemoglobin dissociation curve. The relationship between the Po 2 and the amount of oxygen bound to hemoglobin and the relationship between the Po 2 and the oxygen dissolved in plasma are different. The relationship is S shaped (sigmoid) for hemoglobin; the relationship is linear for plasma. For dissolved oxygen in plasma, the solubility coefficient is 0.003 mL/100 mL at a Po 2 of 1 mm Hg at 37°C (or 0.3 mL of oxygen/100 mL plasma at 100 mm Hg of Po 2 ).
The sigmoid relationship between the Po 2 and the amount of oxygen bound to hemoglobin is expressed by the oxygen-hemoglobin dissociation curve ( Fig. 11-3 ). The Po 2 at which 50% of hemoglobin is saturated has been chosen as the reference point, called P 50 . The P 50 averages 27 mm Hg in adults and 22 mm Hg in fetuses and newborns. The position of the dissociation curve is an expression of the affinity of hemoglobin for oxygen. A newborn’s curve (curve A), with high oxygen affinity, favors the extraction of oxygen from the maternal circulation and suits the conditions of the intrauterine environment, but fetal hemoglobin is “stingy” hemoglobin; it does not allow easy release of oxygen to tissues as in adults. The adult curve (curve B), with a decreased affinity for oxygen, allows the release of more oxygen to tissues. The adult curve is reached by 3 months of age.

The pH, Pco 2 , and erythrocyte concentrations of 2,3-diphosphoglycerate (2,3-DPG), adenosine triphosphate (ATP), MHg, and carboxyhemoglobin influence the position of the dissociation curve (see Fig. 11-3 ).
- 1.
A decrease in hydrogen ion concentration (or increased pH), Pco 2 , temperature, 2,3-DPG, and ATP concentrations shifts the curve to the left (curve A).
- 2.
An increase in the above parameters shifts the curve to the right (curve C).
- 3.
Fetal hemoglobin has considerably less affinity for 2,3-DPS (40%) than does the adult hemoglobin. This makes fetal hemoglobin behave as if 2,3-DPG levels are low, thus shifting the curve to the left.
- 4.
The curve shifts to the right in compensation for high altitude, cyanosis, or anemia as a result of an increase in the red cell concentration of 2,3-DPG.
Consequences and Complications
- 1.
Polycythemia. Low arterial oxygen content stimulates bone marrow through erythropoietin release from the kidneys and produces increased number of red blood cells (RBCs). Polycythemia, with a resulting increase in oxygen-carrying capacity, benefits cyanotic children. However, when the hematocrit reaches 65% or higher, a sharp increase in the viscosity of blood occurs, and the polycythemic response becomes disadvantageous, particularly if the patient has congestive heart failure (CHF). Some cyanotic infants have a relative iron deficiency state, with normal or lower than normal hemoglobin and hypochromia on blood smear. A normal hemoglobin in a cyanotic patient represents a relative iron deficiency state. Although less cyanotic, these infants are usually more symptomatic and improve when iron therapy raises the hemoglobin.
- 2.
Clubbing. Clubbing is caused by soft tissue growth under the nail bed as a consequence of central cyanosis. The mechanism for soft tissue growth is unclear. One hypothesis is that megakaryocytes present in the systemic venous blood may be responsible for the change. In normal persons, platelets are formed from the cytoplasm of the megakaryocytes by fragmentation during their passage through the pulmonary circulation. The cytoplasm of megakaryocytes contains growth factors (e.g., platelet-derived growth factor and transforming growth factor B). In patients with right-to-left shunts, megakaryocytes with their cytoplasm may enter the systemic circulation, become trapped in the capillaries of the digits, and release growth factors, which in turn cause clubbing. Clubbing usually does not occur until a child is 6 months or older, and it is seen first and is most pronounced in the thumb. In the early stage, it appears as shininess and redness of the fingertips. When fully developed, the fingers and toes become thick and wide and have convex nail beds (see Fig. 2-1 ). Clubbing is also seen in patients with liver disease or subacute bacterial endocarditis and on a hereditary basis without cyanosis.
- 3.
CNS complications. Either very high hematocrit levels or iron-deficient RBCs place individuals with cyanotic congenital heart defects at risk for disorders of the CNS, such as brain abscess and vascular stroke. In the past, cyanotic congenital heart diseases accounted for 5% to 10% of all cases of brain abscesses. The predisposition for brain abscesses may partially result from the fact that right-to-left intracardiac shunts may bypass the normally effective phagocytic filtering actions of the pulmonary capillary bed. This predisposition may also result from the fact that polycythemia and the consequent high viscosity of blood lead to tissue hypoxia and microinfarction of the brain, which are later complicated by bacterial colonization. The triad of symptoms of brain abscesses are fever, headache, and focal neurologic deficit.
Vascular stroke caused by embolization arising from thrombus in the cardiac chamber or in the systemic veins may be associated with surgery or cardiac catheterization. Cerebral venous thrombosis may occur, often in infants younger than 2 years of age who have cyanosis and relative iron-deficiency anemia. A possible explanation for these findings is that microcytosis further aggravates hyperviscosity resulting from polycythemia.
- 4.
Bleeding disorders. Disturbances of hemostasis are frequently present in children with severe cyanosis and polycythemia. Most frequently noted are thrombocytopenia and defective platelet aggregation. Other abnormalities include prolonged prothrombin time and partial thromboplastin time and lower levels of fibrinogen and factors V and VIII. Clinical manifestations may include easy bruising, petechiae of the skin and mucous membranes, epistaxis, and gingival bleeding. RBC withdrawal from polycythemic patients and replacement with an equal volume of plasma tend to correct the hemorrhagic tendency and lower blood viscosity.
- 5.
Hypoxic spells and squatting. Although most frequently seen in infants with tetralogy of Fallot (TOF), hypoxic spells may occur in infants with other congenital heart defects (see a later section of TOF for further discussion).
- 6.
Scoliosis. Children with chronic cyanosis, particularly girls and patients with TOF, often have scoliosis.
- 7.
Hyperuricemia and gout. Hyperuricemia and gout tend to occur in older patients with uncorrected or inadequately repaired cyanotic heart defects.
The pathophysiology of individual cyanotic heart defects is discussed in the section to follow.
Common Cyanotic Heart Defects
Complete Transposition of the Great Arteries
Complete transposition of the great arteries (D-TGA) is the most common cyanotic congenital heart defect in newborns, at least in Western countries. In this condition, the aorta arises from the right ventricle (RV), and the pulmonary artery (PA) arises from the left ventricle (LV). As the result, the normal anteroposterior relationship of the great arteries is reversed, so that the aorta is anterior to the PA (transposition) but the aorta remains to the right of the PA; thus, the prefix D is used for dextroposition. In levotransposition of the great arteries (L-TGA, or congenitally corrected TGA), the aorta is anterior to and to the left of the PA; therefore, the prefix L is used (see Chapter 14 ). The atria and ventricles are in normal relationship. The coronary arteries arise from the aorta, as in a normal heart. Desaturated blood returning from the body to the right atrium (RA) flows out of the aorta without being oxygenated in the lungs and then returns to the RA. Therefore, tissues, including vital organs such as the brain and heart, are perfused by blood with a low oxygen saturation. Conversely, well-oxygenated blood returning to the left atrium (LA) flows out of the PA and returns to the LA. This results in a complete separation of the two circuits. The two circuits are said to be in parallel rather than in series, as in normal circulation ( Fig. 11-4 ). This defect is incompatible with life unless communication between the two circuits occurs to provide the necessary oxygen to the body. This communication can occur at the atrial, ventricular, or ductal level or at any combination of these levels.

In the most frequently encountered form of D-TGA, only a small communication exists between the atria, usually a patent foramen ovale (PFO) ( Fig. 11-5 , A ). The newborn is notably cyanotic from birth and has an arterial oxygen saturation of 30% to 50%. The low arterial Po 2 , which ranges from 20 to 30 mm Hg, causes an anaerobic glycolysis, with resulting metabolic acidosis. Hypoxia and acidosis are detrimental to myocardial function. The normal postnatal decrease in pulmonary vascular resistance (PVR) results in increased pulmonary blood flow (PBF) and volume overload to the LA and LV. Severe hypoxia and acidosis (with a resulting decrease in myocardial function) and volume overload to the left side of the heart cause CHF during the first week of life. Therefore, chest radiographs show cardiomegaly and increased pulmonary vascularity. Unless hypoxia and acidosis are corrected, the condition of these infants deteriorates rapidly. Hypoxia and acidosis stimulate the carotid and cerebral chemoreceptors, causing hyperventilation and a low Pco 2 in the pulmonary circulation. Other metabolic problems encountered are hypoglycemia, which is probably secondary to pancreatic islet hypertrophy and hyperinsulinism, and a tendency toward hypothermia. The electrocardiogram (ECG) shows right ventricular hypertrophy (RVH), but RVH may be difficult to diagnose in the first days of life because of the normal dominance of the RV at this age. Usually no heart murmur is noted in a neonate with D-TGA, although a murmur is commonly found in other forms of cyanotic heart defects. The S2 is single, mainly because the pulmonary valve is farther from the chest wall, causing the P2 to be inaudible. A deeply cyanotic newborn with increased pulmonary vascular markings and cardiomegaly without heart murmur can be considered to have TGA until proved otherwise.

The presence of a large atrial septal defect (ASD) is most desirable in infants with TGA. When a large ASD is present, infants have good arterial oxygen saturation (as high as 80% to 90%) because of good mixing ( Fig. 11-5 , B ). Therefore, hypoxia and metabolic acidosis are not the problems in these children. In fact, the idea of the balloon atrial septostomy (Rashkind procedure) was derived from the natural history of infants with TGA and large ASDs. However, the frequency of a large ASD occurring naturally in TGA is low. Infants who have had successful balloon atrial septostomies behave like those with naturally occurring ASDs. As the PVR falls after birth, PBF increases, with an increase in the size of the LA and LV. Although these infants are not hypoxic or acidotic, CHF develops because of volume overload to the left side of the heart. Because the RV is the systemic ventricle, RVH becomes evident on the ECG.
When associated with a large ventricular septal defect (VSD), only minimal arterial desaturation is present, and cyanosis may be missed ( Fig. 11-6 , A ). Therefore, metabolic acidosis does not develop, but left-sided heart failure results within the first few weeks of life as the PBF increases with decreasing PVR. Chest radiographs reflect this, showing cardiomegaly with increased pulmonary vascularity. The ECG may show biventricular hypertrophy (BVH) when the VSD is large: RVH because of the systemic RV and left ventricular hypertrophy (LVH) because of volume overload of the left side of the heart. A heart murmur of VSD is present, and the S2 is single because the P2 is inaudible or pulmonary hypertension is present.

When the VSD is associated with pulmonary stenosis (PS) in infants with TGA, although the VSD helps good mixing, the volume of fully saturated blood returning from the lungs is inadequate (see Fig. 11-6 , B ). Likewise, even after a well-performed Rashkind procedure, the arterial oxygen saturation does not increase much because of the decreased PBF. These infants have severe hypoxia and acidosis and may succumb early in life. This is a good illustration of how the magnitude of PBF affects the arterial oxygen saturation in a given cyanotic congenital heart defect. Because PBF is not increased, the left cardiac chambers are not under increased volume work; therefore, cardiac enlargement and CHF do not develop. Chest radiographs, therefore, show normal heart size and normal or decreased pulmonary vascularity. The ECG shows evidence of BVH; LVH is present because of PS, and RVH is present because of the nature of TGA. Physical examination reveals a PS murmur and a single S2 in addition to cyanosis.
Persistent Truncus Arteriosus and Single Ventricle
In persistent truncus arteriosus ( Fig. 11-7 , A ), a single arterial blood vessel (truncus arteriosus) arises from the heart. The PA or its branches arise from the truncus arteriosus, and the truncus continues as the aorta. A large VSD is always present in this condition. In single ventricle (also called “double-inlet ventricle”) ( Fig. 11-7 , B ), two atrioventricular (AV) valves empty into a single ventricular chamber from which a great artery (either the aorta or PA) arises. The other great artery arises from a rudimentary ventricular chamber attached to the main ventricle. The opening between the single ventricle and the rudimentary chamber is called the “bulboventricular foramen.” No ventricular septum of significance is present (see Fig. 14-61 ).

The following similarities exist between persistent truncus arteriosus and single ventricle from a hemodynamic point of view:
- 1.
There is almost complete mixing of systemic and pulmonary venous blood in the ventricle, and the oxygen saturation of blood in the two great arteries is similar.
- 2.
Pressures in both ventricles are identical.
- 3.
The level of oxygen saturation in the systemic circulation is proportional to the magnitude of PBF.
In addition to the level of PVR, the magnitude of PBF is determined by the caliber of the PA in the case of persistent truncus arteriosus and by the presence or absence of PS and the size of the VSD (i.e., the bulboventricular foramen) in the case of single ventricle. When the PBF is large, the patient is minimally cyanotic but may develop CHF because of an excessive volume overload placed on the ventricle. In contrast, when the PBF is small, the patient is severely cyanotic and does not develop CHF because there is no volume overload. This latter group of patients and those with TOF share similar clinical pictures.
Physical examination reveals varying degrees of cyanosis, depending on the magnitude of PBF. A heart murmur of the VSD is rarely audible because of the presence of a huge defect. There may be an ejection systolic murmur caused by the stenosis of the pulmonary valve or of the PA branches. An early diastolic murmur of truncal valve regurgitation may be heard. The ECG usually shows BVH in both conditions. In single ventricle, the QRS complexes of all precordial leads (i.e., V1–V6) are recorded over one ventricle, and therefore, they are similar (with poor R/S progression), suggestive of BVH. Chest radiographic findings are determined by the magnitude of PBF—if the magnitude of the PBF is large, the heart size is large, and the pulmonary vascularity increases; if the magnitude is small, the heart size is small, and the pulmonary vascularity decreases. With increased PBF and resulting pulmonary hypertension, CHF and later pulmonary vascular obstructive disease (i.e., Eisenmenger’s syndrome) may develop.
Tetralogy of Fallot
The classic description of TOF includes the following four abnormalities: VSD, pulmonary stenosis (PS), right ventricular hypertrophy (RVH), and overriding of the aorta. From a physiologic point of view, TOF requires only two abnormalities—a VSD large enough to equalize systolic pressures in both ventricles and a stenosis of the right ventricular outflow tract (RVOT) in the form of infundibular stenosis, valvular stenosis, or both. RVH is secondary to PS, and the degree of overriding of the aorta varies widely and it is not always present. The severity of the RVOT obstruction determines the direction and the magnitude of the shunt through the VSD. With mild stenosis, the shunt is left to right, and the clinical picture resembles that of a VSD. This is called acyanotic or pink TOF ( Fig. 11-8 , A ). With a more severe stenosis, the shunt is right to left, resulting in “cyanotic” TOF ( Fig. 11-8 , B ). In the extreme form of TOF, the pulmonary valve is atretic, with right-to-left shunting of the entire systemic venous return through the VSD. In this case, the PBF is provided through a patent ductus arteriosus (PDA) or multiple collateral arteries arising from the aorta. In TOF, regardless of the direction of the ventricular shunt, the systolic pressure in the RV equals that of the LV and the aorta (see Fig. 11-8 , A and B ). The mere combination of a small VSD and a PS is not TOF; the size of the VSD must be nearly as large as the annulus of the aortic valve to equalize the pressure in the RV and LV.
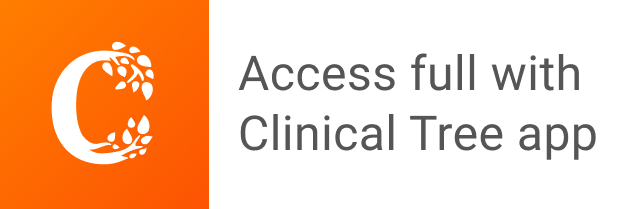