Fig. 59.1
The conotruncal anomalies can be seen as a related group of disorders characterized by an altered rotational relationship between the aorta and the pulmonary artery. (a) Normal heart, (b) TOF, (c) DORV, (d) DORV with malposition/subpulmonic VSD (Taussig-Bing anomaly), and (e) d-TGA
A number of related developmental abnormalities may produce obstructive lesions on the left side of the heart. These include bicuspid aortic valve (BAV), congenital mitral stenosis, and coarctation of the aorta (CoA). Defects in blood flow through the left side of the heart are thought to produce abnormalities in growth of the left ventricle. In its most severe form, this phenomenon is referred to as hypoplastic left heart syndrome (HLHS). Typically, obstruction of blood flow causes the proximal aorta and arch to be small, and deoxygenated blood in the pulmonary artery enters the systemic circulation through a patent ductus arteriosus (PDA).
There are several other ways to classify CHD: alphabetical order, cyanotic and acyanotic, site of the defect (veins, atria, ventricles, septa, and great arteries), embryologic origin of the defects, etc. [15–17]. However, a pathophysiological classification, namely, a classification based upon the clinical consequences of structural defects impairing the physiology of blood circulation [18], may be more helpful for understanding the clinical presentation and anticipating the long-term consequences of these lesions and thus for planning of appropriate pharmacotherapy. Pathophysiologically, these defects can be categorized into five types as listed in Table 59.1.
Table 59.1
Pathophysiological classification of congenital heart defects
I. Acyanotic CHD with increased pulmonary blood flow (septal defects without pulmonary obstruction and left-to-right shunt) | II. Cyanotic CHD due to right-to-left shunt |
Partial anomalous pulmonary venous drainage | Pulmonary valve stenosis/atresia with ASD |
Atrial septal defects (ASD) | Pulmonary valve stenosis/atresia with VSD (tetralogy of Fallot) |
Complete atrioventricular septal defect | Ebstein anomaly of the tricuspid valve with interatrial communication |
Ventricular septal defects (VSD) | Double-outlet right ventricle |
Persistent truncus arteriosus, aortopulmonary window, patent ductus arteriosus | Persistent truncus arteriosus communis |
Eisenmenger syndrome | |
III. Cyanotic CHD due to admixture of pulmonary and systemic venous blood | IV. CHD incompatible with postnatal blood circulation |
Tricuspid atresia | Parallel systemic and pulmonary circulations (complete transposition of great arteries) without interatrial communication |
Total anomalous pulmonary venous connection with interatrial communication | Total anomalous pulmonary venous drainage without interatrial communication |
Univentricular atrioventricular connections (double-inlet left ventricle) | Ductus-dependent CHD (pulmonary atresia, aortic and mitral atresia, and interrupted or atretic aortic arch) |
V. Acyanotic CHD without shunt | |
Bicuspid aortic valve | |
Pulmonic stenosis | |
Coarctation of the aorta | |
Congenital anomalies of the coronary arteries | |
Congenitally corrected transposition of the great arteries |
Patients in group I are acyanotic at birth, but the increased pulmonary blood flow may result in damage of the small pulmonary arteries and arterioles, leading to pulmonary vascular disease and increased pulmonary resistance. This ultimately reverses the direction of the shunt, converting it from a left-to-right shunt into a right-to-left shunt. When the shunt reverses, cyanosis occurs, resulting in the Eisenmenger syndrome. On the other hand, patients in groups II and III are cyanotic at birth due to right-to-left shunt or to mixing of systemic and pulmonary venous blood and have variable pulmonary blood flow. This is a heterogeneous group, but their clinical presentation and their long-term outcomes are influenced markedly by the presence and/or development of pulmonary vascular disease later in life.
59.3 Pathophysiology of Congenital Heart Disease
59.3.1 Developmental Genetics of CHD
Although epidemiological data point to environmental influences like drugs, toxins, etc., as risk factors for developing CHD [17], the observation of frequent familial recurrence of defects suggests that genetic factors are at play in the development of cardiac defects. Genetic animal models have identified a core group of regulatory genes involved in cardiac development. Chief among these are several key transcription factors (e.g., nkx2-5, gata4, mef2c, tbx5), along with their transcriptional targets, and signaling molecules (particularly fibroblast growth factors, bone morphogenetic proteins, hedgehog, notch, and retinoic acid). These studies have suggested the possibility that disruptions in these genes may result in congenital heart defects, and indeed, several clinical reports have identified mutations in these loci in patients with structural CHD [19]. The earliest cardiac progenitors arise from lateral plate mesoderm, controlled by a cascade of interacting transcription factors. Additional inputs come from secreted molecules, such as fibroblast growth factors, bone morphogenetic proteins, Wnt proteins, and others [19]. The discovery of a “second” heart field (SHF) has led to new understanding of the origin and patterning of the embryonic heart [20]. The SHF is medial and dorsal to the early differentiating cardiomyocytes that comprise the “cardiac crescent” and give rise to a large portion of the heart, including the outflow tract, right ventricle, and parts of the atria. The SHF is further subdivided into a number of lineage pools, which contribute either to anterior structures (such as the outflow tract) or posterior components (such as the atria) [20]. These findings help explain how mutations affecting specific cell lineages within the SHF result in a spectrum of complex defects in heart structures. Altered hemodynamics, signaling defects leading to valve disease, and misregulated expression and function of microRNAs have also recently been implicated as mechanisms for the development of CHD in humans [21]. See also Chap. 62 on miRNAs in cardiovascular development.
Remarkable strides have been made in recent years in the field of cardiac development, particularly in dissecting the transcriptional network (e.g., involving the transcription factors TBX5, NKX2-5, GATA4, TBX1, SALL4, TBX20, TFAP2B, and THRAP2) [21–34], and recognizing that these proteins participate in a complex set of interactions has been important for understanding the regulation of cardiac gene expression and cardiac development. These discoveries have also provided some insight into the etiology of human congenital heart diseases and their patterns of inheritance. However, our understanding of the genetics of CHD is currently incomplete, and the mechanisms of how molecular defects in these transcriptional pathways translate to a structural defect need to be explored further.
59.3.2 Physiological Consequences of CHD
Although the structural variations seen in congenital heart disease constitute an encyclopedic list of complex malformations, these defects can be understood in a more limited physiologic spectrum as described in Table 59.1. Defects in each category impose three basic pathophysiologic burdens on the cardiovascular system: pulmonary overcirculation with ventricular volume overload, ventricular pressure overload, and hypoxemia. Ultimately, these pathophysiologic conditions can result in myocardial failure or pulmonary vascular disease. Medical and surgical management strategies are thus generally focused on minimizing the pathophysiologic consequences of these lesions. However, since CHD is a developing field, future studies are needed to develop specific strategies for management of these pathophysiological consequences. Pulmonary vascular disease, which is a major driver of long-term outcomes in most of the complex congenital heart lesions, is one of the only areas in ACHD where randomized controlled data regarding pharmacotherapy are available, and thus this topic will be the focus of the remainder of this chapter.
59.4 Pulmonary Vasculature
The normal pulmonary circulation differs dramatically from the systemic circulation in its biophysical and hemodynamic properties, structural organization, and physiology. While the systemic circulation is designed to deliver nutrients and oxygen to the tissues, the pulmonary circulation is designed to maximize oxygen uptake in the blood that will ultimately nourish the rest of the body. In order to maximize gas exchange, the pulmonary circulation has an extremely high capacitance, receiving 100 % of cardiac output during each cardiac cycle. Despite the expansive volume of blood present, the pulmonary circulation maintains a low pressure and a low resistance, allowing the right heart to effectively and efficiently pump this large volume of blood through the pulmonary vascular circuit.
59.4.1 Development of the Pulmonary Vascular Bed
The human intrapulmonary arterial branching system develops by the continuous expansion of the mesenchymal primary capillary plexus [35]. The preacinar intrapulmonary arteries are formed primarily by vasculogenesis, a coalescence of cells derived from the mesenchyme into endothelial tubes. During development, endothelial tubes are continuously added at the lung periphery. These arteries increase in size with age and become invested with smooth muscle cells that also mature with age judged by their cytoskeletal protein expression. Thus, the older, more proximal arteries have a more mature cytoskeleton than do the peripheral arteries during development, but by birth, all show a similar expression of proteins. The pulmonary arterial smooth muscle cells seem to originate from more than one source (bronchial smooth muscle cells surrounding the airway, lung mesenchyme, and endothelial cells), but all appear to follow the same pattern of maturation, expressing the same cytoskeletal proteins sequentially in utero [35].
59.4.2 Pathophysiology of Pulmonary Vascular Disease
Pulmonary arterial hypertension (PAH) is a dynamic and multifactorial process related to vasoconstriction and remodeling of the pulmonary vascular bed that is aggravated and accelerated by thrombosis [36]. Several histopathological abnormalities associated with PAH due to congenital shunt lesions (PAH-CHD), such as extension of smooth muscle cells into peripheral pulmonary arteries, medial hypertrophy, formation of plexiform lesions, and rarefaction of the pulmonary arterial tree, have been described [36–38]. Reflecting these histological changes, classifications of pulmonary vascular changes have been developed, including those introduced by Heath and Edwards in 1958 [38] and Rabinovitch in 1978 [39]. It has been suggested that these histological changes may correlate with clinical severity of PAH [40]. In addition to histological studies in patients with PAH, several animal models of congenital heart disease have been established [41, 42] and have provided additional insights into the pathophysiology of the disease (Chap. 45).
The classical model of the pathophysiology of pulmonary vascular disease related to congenital shunt lesions has focused on the notion that high flow and pressure induce pulmonary vascular endothelial damage, leading to a loss of endothelial barrier function [43, 44]. This may be associated with degradation of extracellular matrix (activation of endogenous vascular elastase and matrix metalloproteinases) as well as release of growth factors (fibroblast growth factor and transforming growth factor-β) [45]. In turn, these factors induce smooth muscle cell hypertrophy and proliferation, resulting in extension of smooth muscle cells into peripheral pulmonary vasculature and smooth muscle cell migration with neointima formation [46, 47]. Furthermore, endothelial damage may result in adhesion and activation of platelets and leukocytes, resulting in an inflammatory response, as well as thrombosis and activation of coagulation pathways [36]. Activation of platelets and the coagulation cascade is thought to reinforce the inflammatory reaction in the pulmonary endothelium and proliferative reactions in the smooth muscle [48]. The extent to which inflammation plays a role in the pathogenesis of PAH-CHD is not yet known. However, several studies have shown that markers of inflammation correlate with disease activity and prognosis [49].
In the classical model, high pulmonary blood flow, particularly as a consequence of high-pressure, post-tricuspid shunts, results in endothelial dysfunction, characterized by dysregulation of several signaling pathways involved in control of pulmonary vascular tone and proliferation of pulmonary vascular smooth muscle. Chief among these are nitric oxide (NO) signaling pathway, the prostacyclin signaling pathway (including prostaglandin I2 and thromboxane), and the endothelin signaling pathway [50]. These factors normally maintain a dynamic equilibrium between vasoconstriction and vasodilation that regulates pulmonary vascular resistance to meet metabolic demands. The changes in these signaling processes shift the balance in favor of factors inducing vasoconstriction and ultimately pulmonary vascular remodeling [50]. This complex process of endothelial dysfunction forms the basis of our current approach to treatment, and these altered signaling pathways are the targets of “advanced therapy.”
Different mediators influencing pulmonary vascular tone have been identified, some of which are currently amenable to pharmacological therapy. Extensive activation of the endothelin system is one of the hallmarks of PAH and is likely to contribute to pulmonary vasoconstriction and vascular remodeling [43, 44]. In addition, circulating endothelin levels have been found to correlate with disease severity and outcome in PAH patients [45]. Decreased production of prostacyclin is an additional feature of PAH. Prostacyclin, a metabolite of arachidonic acid, is a potent pulmonary and systemic vasodilator [51]. In patients with PAH, prostacyclin production is impaired, and levels of prostacyclin metabolites are reduced [52, 53]. Nitric oxide, a potent endothelium-derived factor inducing vasodilation and suppressing proliferation, is involved in the pathophysiology of PAH [52, 53]. Nitric oxide activates cyclic guanylyl cyclase in vascular smooth muscle cells, leading to increased intracellular levels of cGMP; cGMP in turn is degraded by phosphodiesterases. Pharmacological inhibition of phosphodiesterases provides a way to induce cGMP-dependent vasodilation (see Chap. 31).
The classical view of endothelial dysfunction is clearly incomplete as a framework for advanced therapy, and further studies are needed in order to develop a more complete understanding of the pathogenesis of PAH-CHD. Indeed, preliminary data suggest additional mechanisms at play in the development of PAH-CHD. First, there are differences in the risk of developing pulmonary vascular disease when one compares different lesions with the same pulmonary blood flow and initial pulmonary pressures. This suggests the possibility that these defects may differ in terms of underlying genetic susceptibility. Much focus of genetic studies has been on mutations that affect transforming growth factor-β (TGF-β) superfamily signaling. Indeed, mutations in bmpr2, which encodes the bone morphogenetic protein (BMP) type II receptor, and alk1, which encodes activin-like kinase type 1, have been implicated in both familial PAH and in up to 6 % of patients with PAH-CHD [54–57].
Additional abnormalities have been described in PAH associated with congenital heart disease that might provide new insights into pathophysiology and also may have future therapeutic implications. These include increased turnover of serotonin, a pulmonary vasoconstrictor, compared with healthy individuals [58] and emerging evidence of altered intrapulmonary expression of TGF-β1 and its receptors in an animal model of shunt-induced pulmonary vascular disease [54]. Furthermore, altered expression of pulmonary potassium channels associated with an accentuated response to hypoxia has been shown in an ovine model [55].
These preliminary data reflect the complex interplay between genetic susceptibility and environmental factors such as pulmonary blood flow or pressure in the etiology of PAH associated with congenital heart disease.
59.5 Pharmacotherapy for Pulmonary Vascular Disease Associated with Congenital Heart Disease
In the European guidelines, PAH-CHD patients have been classified into four main clinical groups (Table 59.2) [59]. Current guidelines recommend that CHD patients requiring PAH-specific therapy be managed in specialized centers. Although some data on the use of PAH-specific therapies are available, particularly for Eisenmenger syndrome patients, guidelines are generally based on the clinical experience of experts rather than formal evidence from clinical trials [59].
Table 59.2
Clinical classification of congenital, systemic-to-pulmonary shunts associated with pulmonary arterial hypertension (PAH)
Group A. Eisenmenger syndrome |
Eisenmenger syndrome includes all systemic-to-pulmonary shunts due to large defects leading to a severe increase in PVR and resulting in a reversed (pulmonary-to-systemic) or bidirectional shunt. Cyanosis, erythrocytosis, and multiple-organ involvement are present |
Group B. PAH associated with systemic-to-pulmonary shunts |
In these patients with moderate-to-large defects, the increase in PVR is mild to moderate, systemic-to-pulmonary shunt is still largely present, and no cyanosis is present at rest |
Group C. PAH with small defects |
In cases with small defects (usually VSD <1 cm and ASD <2 cm of effective diameter assessed by echocardiography), the clinical picture is very similar to idiopathic PAH |
Group D. PAH after corrective cardiac surgery |
In these cases, CHD has been corrected, but PAH is either still present immediately after surgery or has recurred several months or years after surgery in the absence of significant postoperative residual congenital lesions or defects that originate as a sequelae to previous surgery |
59.5.1 General Management and Background Therapy
General measures for the treatment of patients with PAH-CHD and, in particular, those with Eisenmenger syndrome include recommendations for physical activity, prevention of dehydration, infections, air travel, exposure to high altitudes and elective surgery, and that psychological assistance be provided as necessary [60]. Patients with Eisenmenger syndrome are at particular risk during anesthesia and surgery, and special care is required. Pregnancy is contraindicated in patients with Eisenmenger syndrome, as there is a high risk of maternal and fetal mortality; adequate contraception is therefore mandatory. Patients with Eisenmenger syndrome are at an increased risk of coagulation disorders. The high incidence of pulmonary artery thrombosis (up to 20 %) is associated with increasing age, biventricular dysfunction, and dilatation of the pulmonary arteries. However, the use of anticoagulants in this population is controversial as there is also an increased risk of hemoptysis and hemorrhage [10]. Routine laboratory-guided phlebotomy should not be performed as secondary erythrocytosis is beneficial for oxygen transport and delivery [61]. If moderate-to-severe symptoms of hyperviscosity are present, and iron deficiency and dehydration have been excluded, phlebotomy with isovolumic replacement should be performed carefully when the hematocrit is >65 % [10]. Iron deficiency has been shown to be associated with a higher risk of adverse outcomes (all-cause mortality, transplantation, and hospitalization due to cardiopulmonary causes) in Eisenmenger syndrome patients, and iron replacement therapy improves exercise tolerance and quality of life [61, 62]. However, care should be taken to avoid an excessive rise in hemoglobin concentration and decompensated polycythemia [61].
One of the first classes of drugs to be studied for the treatment of patients with PAH are the calcium channel blockers (CCBs). In one early study, 64 patients with iPAH were treated with nifedipine or diltiazem, and those who had a hemodynamic response were treated for 5 years. This subgroup was found to have improved survival compared with those who did not respond. While this finding may suggest a role for CCBs in patients with PAH-CHD, the systemic vasodilatory effect of these agents may result in worsening of the right-to-left shunt, thereby posing a risk to the patient. There are no data from clinical trial to support the use of CCBs in patients with PAH-CHD, and the current guidelines suggest that their use be avoided [59]. In fact, their use is contraindicated in Eisenmenger syndrome patients as their vasodilatory effect can result in an acute decrease in systemic vascular resistance and therefore an increase in right-to-left shunting, which may lead to syncope and sudden death [63]. Embolization therapy of collateral vessels may be helpful in the management of selected patients with acute significant hemoptysis but must be weighed against the risk associated with the consequent reduction in the cross-sectional area of the pulmonary vascular bed [59]. The use of supplemental oxygen therapy is controversial, and it should be used only in whom it produces a consistent increase in arterial oxygen saturation [59].
59.5.2 Management of PAH-CHD with PAH-Specific Therapies
In the past two decades, multiple randomized controlled studies [64–91] have demonstrated the efficacy of three classes of drugs or their combination for the treatment of PAH (see Table 59.3), namely, (i) prostanoids, (ii) endothelin receptor antagonists. and (iii) phosphodiesterase (PDE)-5 inhibitors (see also Chap. 45). The rationale supporting the use of these drugs is based on targeting the mediators of endothelial dysfunction [92, 94]. Most randomized trials of pulmonary vasodilators have included mostly patients with idiopathic PAH, with only a small percentage of PAH-CHD patients. The clinical database is therefore limited.
Table 59.3
Pharmacotherapy of pulmonary arterial hypertension
Agents | Route of administration | Dose | Half-life | Adverse effects |
---|---|---|---|---|
Prostanoids | ||||
Epoprostenol | Continuous intravenous | 1–2 ng/kg/min initially Increased by 1–2 ng/kg/min every 1–2 weeks until therapeutic response or dose-limiting toxicity occurs No maximal dose established | 3–5 min (single dose) 15 min (continuous infusion) | Jaw pain, diarrhea, flushing, and arthralgias. Excess dose may cause high-output cardiac states Adverse effects related to the intravenous pump |
Treprostinil | Intravenous, subcutaneous (used less often) | 0.625–1.25 ng/kg/min initially Dose titrated up every 1–2 weeks until therapeutic response or dose-limiting toxicity occurs | 4 h | Same as above |
Inhaled | One to three inhalations (i.e., 6–18 μg), four times daily initially Maintenance dose may be gradually titrated up to nine inhalations (i.e., 54 μg), four times daily | 4 h | ||
Iloprost | Inhaled | 2.5–5 μg, six to nine times daily | 20–30 min | Main disadvantage is the need for frequent administration |
Endothelin receptor antagonists | ||||
Bosentan | Oral | 62.5–125 mg, two times daily | 5 h | Hepatotoxicity, peripheral edema, teratogenic |
Dose is adjusted for low body weight or drug interactions | ||||
Ambrisentan | Oral | 5–10 mg daily | 9 h | Peripheral edema, worsening of idiopathic pulmonary fibrosis, teratogenic, least hepatotoxic among ERAs |
Macitentan | Oral | 3–10 mg per day | 14–18 h | Nasopharyngitis, anemia, teratogenic, edema less common |
Phosphodiesterase type 5 inhibitors | ||||
Sildenafil | Oral | 20 mg, three times daily | 4 h | Flushing, diarrhea, headache, myalgia, and visual disturbances |
Intravenous | 10 mg, three times daily Dose is adjusted for drug interactions | 4 h | ||
Tadalafil | Oral | 40 mg daily Dose is adjusted for drug interactions | 35 h | |
Soluble guanylyl cyclase stimulant | ||||
Riociguat | Oral | 0.5–1 mg three times daily initially Titrated up by 0.5 mg three times per day every 2 weeks until therapeutic response or dose-limiting toxicity occurs (maximum dose 2.5 mg three times daily) | 12 h | Syncope |
Prostanoids – Prostanoid formulations used to treat PH include intravenous epoprostenol (prostacyclin), intravenous treprostinil, subcutaneous treprostinil, inhaled treprostinil, and inhaled iloprost [51, 93]. The clinical utility of all of these agents is limited by the fact that they must be administered parenterally.
Epoprostenol – Intravenous epoprostenol is the advanced therapy that has been best studied. It improves hemodynamic parameters, functional capacity, and survival in patients with iPAH [94, 95]. However, survival has not been adequately evaluated due to limited sample sizes [96]. Data supporting the use of epoprostenol in patients with PAH-CHD come primarily from uncontrolled case series. In one retrospective study of 20 PAH-CHD patients who had failed conventional therapy with diuretics, digitalis, and warfarin, chronic treatment with intravenous epoprostenol was associated with a long-term improvement in pulmonary hemodynamics, functional class, and exercise tolerance. A later retrospective study confirmed these clinical and hemodynamic improvements in patients treated with intravenous epoprostenol [98].
Treprostinil – Treprostinil can be given intravenously or subcutaneously, although subcutaneous administration is used less often due to severe pain at the injection site. Inhaled treprostinil has more recently been approved for patients who are WHO functional class III. Intravenous and subcutaneous treprostinil improves hemodynamic parameters, symptoms, exercise capacity, and possibly survival [70, 93, 97, 99]. Advantages of parenteral treprostinil, compared to epoprostenol, include the option of continuous subcutaneous delivery, a longer half-life that may make interruption of the infusion less immediately life threatening, and no need for refrigeration. Based on the results of two clinical trials, an oral formulation of treprostinil was approved by the US Food and Drug Administration (FDA) [81, 82]. The benefits of oral treprostinil in these studies were small although statistically significant. As with epoprostenol, there are no prospective, randomized controlled trials of treprostinil in PAH-CHD patients. However, a retrospective study suggested benefit in these patients.
< div class='tao-gold-member'>
Only gold members can continue reading. Log In or Register a > to continue
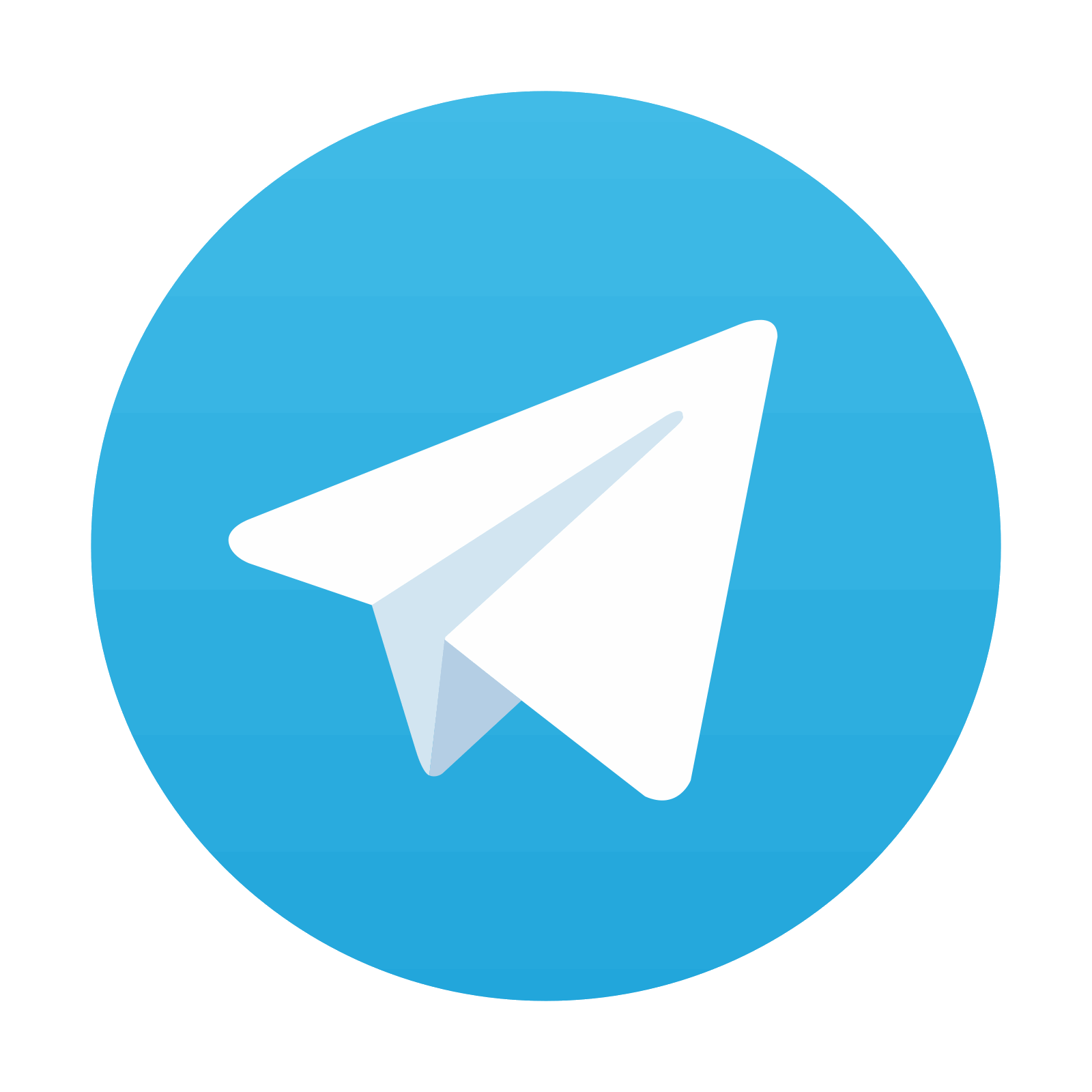
Stay updated, free articles. Join our Telegram channel
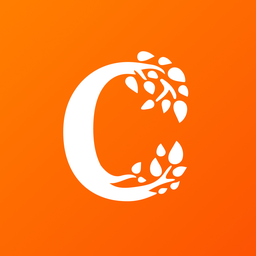
Full access? Get Clinical Tree
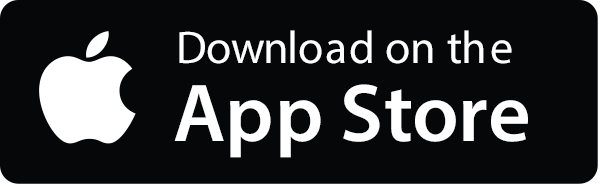
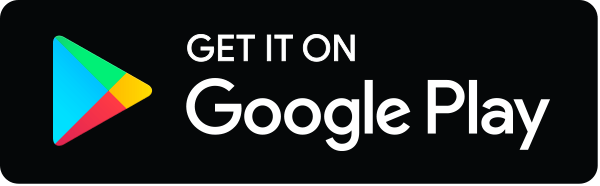