Fig. 4.1
(a) Panel A. From a newborn infant 4 days old. The intima is the indistinct layer lying upon the internal elastic membrane (I). Elastic fibers are distributed irregularly in media (M). The adventitia (A) is thick and collagenous. Elastic tissue stain ×170. Panel B. From a 3-month-old infant. Image shows focal thickening of the wall by formation of the musculoelastic layer. Elastic tissue stain ×40. (b) Coronary arteries in male infants from three ethnic groups. Panel A. There are differences in the intensity and quantity of the structural findings between the sexes and among various ethnic groups in early life. Panel B. Mean values of measurements of the intima and musculoelastic layer in coronary arteries in males of three ethnic groups (Adapted from Vlodaver Z, Kahn HA, and Neufeld HN. The coronary arteries in early life. Circulation. 1969; 39:541 [1]) Elastic tissue stain ×40
A normal variation, which may be observed even in the young, includes an extra layer between the intima and media. This is a musculoelastic layer, characterized by a focal aggregation of smooth muscle and connective tissue (◘ Fig. 4.1a B).
Coronary Vascular Changes in Childhood
Intimal Thickening
The earliest vascular change described microscopically is intimal thickening, which consists of layers of smooth muscle-like cells and an extracellular matrix. Segments of the media related to the musculoelastic layer may show thinning.
Thickening of the intima in childhood may relate to later development of coronary artery disease.
In 1969, we described the histological changes in the coronary arteries in full-term fetuses, infants, and children of 211 consecutive necropsy specimens from Ashkenazy, Yemenite, and Bedouin groups [1]. We found differences in early life in the intensity and quantity of the structural findings between the sexes and among various ethnic groups (◘ Fig. 4.1b A and B).
These differences between sexes and ethnic groups in children up to 10 years old were consistent with the known differences in the prevalence of coronary atherosclerosis, coronary heart disease, and myocardial infarction in the corresponding adult population.
Intimal Fatty Streak
Intimal fatty streaks are lesions primarily composed of abundant macrophage foam cells interspersed with smooth muscle cells. Although this entity is referenced as the earliest of atherosclerotic lesions, in most cases its development is a reversible process with no progressive tendency. Many studies describe the complete regression of the intimal fatty streak [2, 3].
In 2000, based on morphologic characterizations, Virmani proposed a modified American Heart Association (AHA) Consensus Classification of coronary atherosclerotic lesions with emphasis on the relationship between atheromatous progression and acute coronary syndromes, grouping the lesions without and with thrombosis [4].
Atherosclerotic Lesions Without Thrombosis
Pathologic Intimal Thickening
The earliest of progressive lesions is called pathologic intimal thickening . These lesions are mainly composed of layers of smooth muscle cells aggregated near the lumen with an underlying lipid pool existing as a relatively acellular area rich in hyaluronan and proteoglycans.
Fibrous Cap Atheromas
Fibrous cap atheromas are characterized by an acellular necrotic core which is contained by an overlying layer of fibrous tissue forming a distinct entity referred to as the “fibrous cap” (◘ Fig. 4.2a). It is distinguished from lipid pool lesions of pathologic intimal thickening and represents a further progressive stage of atherosclerotic disease [5].


Fig. 4.2
(a) Fibrous cap atheroma characterized by an acellular necrotic core which is contained by an overlying layer of fibrous tissue. This forms a distinct entity referred to as the “fibrous cap.” (b) Thin-cap fibroatheroma with hemorrhage in atheroma exhibits large necrotic cores with overlying thin, intact fibrous caps infiltrated by macrophages. (c) Plaque rupture . Disruption of the fibroatheroma cap, and a luminal thrombus communicates with the underlying necrotic core. (d). Plaque erosion. The plaque structure is similar to the plaque in cases with rupture, but with no communication of the thrombus with the necrotic core
In the early stages, a focal macrophage infiltrates into areas of lipid pools, and, in later stage of necrosis, a loss of matrix occurs with resultant extensive cellular debris. The fibrous cap is critical to maintaining the integrity of the lesion and is subject to thinning prior to the onset of rupture.
Thin-Cap Fibroatheroma
Thin-cap fibroatheromas (TCFAs) are lesions that exhibit large necrotic cores with overlying thin, intact fibrous caps infiltrated by macrophages (◘ Fig. 4.2b).
Thin-cap fibroatheromas, traditionally referred to as vulnerable plaques, morphologically resemble ruptured plaque, although they are discriminated by the lack of a luminal thrombus and disrupted fibrous cap. Typically, few or no smooth muscle cells are present within a fibrous cap.
Vulnerable plaque is a term that represents “the susceptibility of a plaque to rupture.” Studies of autopsies of patients who had died of cardiac causes show that the most common underlying plaque morphology was a ruptured thin-cap fibroatheroma with superimposed thrombosis [6].
This pathophysiological background spurred interest in identifying such vulnerable plaques in stable patients. The ultimate goal is preventing plaque rupture, thereby averting myocardial infarction and sudden death [7].
Narula et al. [8] compared stable plaques, thin-cap fibroatheromas, and ruptured caps in sudden death victims. They found that fibrous cap thickness was the best discriminator of plaque type, measuring <54 μm in ruptured plaques, 54–84 μm in most TCFAs, and >84 μm in stable plaques. In this pathologic study, the authors also observed that the incidence of myocardial infarctions tended to emerge from previously obstructed lesions, the TCFA exhibited >50 % stenosis, and majority of ruptured plaques showed >75 % stenosis.
Atherosclerotic Lesions Associated with Acute Thrombosis
Plaque Rupture
With plaque rupture , we see disruption of the fibroatheroma cap and the luminal thrombus communicating with the underlying necrotic core (◘ Fig. 4.2c).
The fibrous cap consists mainly of type I collagen with varying degrees of macrophages and lymphocytes and very few, if any, alpha-actin-positive smooth muscle cells. The luminal thrombus often is platelet-rich near the actual rupture site, giving a white appearance (white thrombus). In contrast, near the rupture site and at sites of propagation, the luminal thrombus is composed of layers of fibrin and red blood cells (red thrombus) seen at the proximal and distal ends of the thrombus.
Rupture of an atherosclerotic plaque had been uniformly accepted as the primary causative event in sudden coronary death. Although the underlying mechanisms of plaque rupture are poorly understood, several critical processes including matrix degradation by matrix metalloproteinases (MMPs), high shear stress regions, stress branch points, macrophage death, and microcalcification and iron accumulation within the fibrous cap are thought to play a role [9].
Plaque Erosion
Several studies of patients who died due to acute myocardial infarction (AMI) or sudden death described superficial erosion of plaque as an important substrate for coronary thrombosis, usually nonocclusive.
This plaque structure is similar to the plaque in cases with rupture, but there is no communication of the thrombus with the necrotic core (◘ Fig. 4.2d).
Serial sectioning confirms that, with plaque erosion, the thrombus is confined to the luminal plaque surface with an absence of fissures or communication with an underlying necrotic core. The term “erosion” is used because the luminal surface beneath the thrombus lacks endothelial cells [10].
Intimal Calcification
The extent of coronary artery calcification correlates with plaque burden. Calcified nodules might disrupt the fibrous cap, leading to thrombosis [6]. Recurrent plaque rupture and hemorrhage with subsequent healing might result in the development of obstructive fibrocalcified lesions and are frequently found in patients with stable angina and sudden coronary death.
Intravascular ultrasound (IVUS) has played an important role in understanding the pathology and treatment of atherosclerosis in humans. Several grayscale IVUS features have been associated with either clinical instability or a high risk for cardiovascular events in patients with coronary artery disease who undergo percutaneous coronary intervention (◘ Fig. 4.3) [11].


Fig. 4.3
Intravascular ultrasound of a coronary segment with large atherosclerotic plaque . Using “virtual histology” analysis of the plaque composition, the plaque is composed of fibrofatty tissue (green) and necrotic core (red). The white areas represent calcification
Stem Cells and Atherosclerosis
A variety of mechanisms contributes to the development and progression of atherosclerosis , including genetic and immunologic mechanisms, hemodynamic effects, and risk factors both known and unknown. However, a principal initiator of atherosclerosis appears to be the development of endothelial dysfunction following arterial injury.
Circulating stem cells and progenitor cells derived from the bone marrow and vasculature play critical roles in vascular repair and homeostasis and may serve to counteract the development of atherosclerosis following vessel injury [12].
Since the initial observation that circulating human CD34+/KDR+ mononuclear cells assume an endothelial phenotype in culture and incorporate into newly formed vasculature in the ischemic hind limb, a new paradigm for postnatal vasculogenesis was discovered [13]. Multiple studies have confirmed the importance of these putative endothelial progenitor cells (EPCs) and other bone marrow-derived cells in promoting vascular health. This is supported, in part, by the relationship in many studies between the number of circulating EPCs and the development of atherosclerosis [14].
Paradoxically, stem cells and progenitor cells may also contribute to the progression of atherosclerosis. The development of transgenic animals that develop atherosclerosis—for example, apolipoprotein E knockout (ApoE−/−) mice—or express markers on bone marrow-derived cells, green fluorescent protein (GFP), has provided new insight into how vascular and bone marrow-derived stem cells and progenitor cells are involved in the biology of atherosclerosis.
Recent investigation has shown that bone marrow-derived EPCs and smooth muscle progenitor cells (SPCs) may play important roles in the biology of atherosclerosis and plaque rupture. EPCs may increase vascularization of the plaque, which may increase the likelihood of plaque rupture [14], while SPCs may increase plaque stability [15]. These seemingly contradictory roles of stem and progenitor cells in atherosclerosis are the subject of ongoing investigation.
Acute Myocardial Infarction
Coronary syndromes that cause a relatively rapid onset or increase of symptoms or ischemia are termed acute coronary syndromes (ACSs) . An ACS is a continuum of unstable coronary syndromes that stretches from “unstable angina” to acute ST segment elevation myocardial infarction (STEMI) .
Acute myocardial infarction (MI) occurs when there are severe reductions in coronary blood flow and myocardial delivery for more than 20 min. The infarct begins in the subendocardium (inner or mid wall of the heart) and is confined in the first 30 min to 2 h. If the artery thrombosis is transient or does not cause complete coronary artery occlusion, an infarct to the subendocardium infarction develops, also described as non-Q or non-ST segment elevation myocardial infarction (NSTEMI) . If the coronary artery occlusion is sustained for longer periods, the myocardial necrosis progresses toward the epicardium in the next 2–3 h, and a “Q wave” or STEMI transmural infarction develops.
The role of coronary artery thrombosis in causing MI was debated until studies by DeWood and colleagues in 1980 demonstrated by coronary arteriography that coronary thrombosis is virtually always the cause of acute Q wave or STEMI [16]. Ninety percent or more of STEMIs have prolonged occlusive coronary artery thrombosis in the infarct-related coronary artery [17].
Only about 30 % of patients with NSTEMI have an occlusive thrombus in the infarct-related artery. In most patients with NSTEMI, transient coronary artery occlusion is initiated by platelet aggregation and associated vasoconstriction is present. Distal embolization, the cause for the rise in biomarkers, is frequently present with NSTEMI .
Local accumulation of endothelium causes marked vasoconstriction. Serotonin, adenosine diphosphate, thrombin, and endothelin are mitogens, and they likely contribute to subsequent local fibroproliferation with increases in the neointima, with further narrowing of the lumen of the endothelium-injured coronary artery. Reduction in fibrinolytic capability at sites of vascular endothelium injury is associated with decreases in vascular tissue concentration of protacyclin, tissue plasminogen-activating factor, and nitric oxide. This contributes to coronary artery thrombosis, vasoconstriction, and fibroproliferation at these sites [18].
The process of NSTEMI and STEMI begins with coronary endothelium injury, usually atherosclerotic plaque ulceration or fissuring. The degree of coronary artery stenosis where plaque ulceration or fissuring occurs may be mild or severe. About half of the coronary stenosis where plaque fissuring or ulceration occurs are sites of less than 50 % normal luminal diameter [19].
Fissuring and ulceration of the plaque often occur in the asymmetric portion or “shoulder region” with a thin fibrous cap that is lipid laden. Inflammation at the sites of these fibrous plaques with adjacent lipid cores best predicts the vulnerable atherosclerotic plaque and one likely to fissure or ulcerate [20].
Inflammation is characterized in the unstable plaque by the accumulation of monocyte-derived macrophages, activated T cells, and mast cells. Most likely, proteases released from the infiltrating mononuclear cells contribute to thinning of the fibrous cap through their degradation of collagen and subsequent atherosclerotic plaque fissuring and ulceration [20].
Pathologic Features
Myocardial infarcts less than 12 h old usually are not grossly apparent. However, infarcts more than 3 h old can be visualized by exposing myocardium to vital stains, such as triphenyltetrazolium chloride, a substrate for lactate dehydrogenase. Because ischemic necrosis causes the enzyme to leak out of damaged cells, the infarcted area is unstained (pale), while old scars appear white and glistening.
At about 12 h postinfarct, the involved myocardium may grossly reveal a bluish hue. Histologically, the involved myocardial fibers may be somewhat more eosinophilic than fibers in the uninvolved areas of the ventricle.
By 24 h, the bluish hue gives way to a slightly tan shade. Histologically, there may be some clumping of the cytoplasm of the myocardial fibers. The capillaries tend to be dilated, and early interstitial exudation of leukocytes, predominantly neutrophils, takes place, and the fibers appear more eosinophilic (◘ Fig. 4.4a).


Fig. 4.4
(a) By 24 h, there is clumping of the cytoplasm of the myocardial fibers, the capillaries tend to be dilated, and early interstitial exudation of leukocytes, predominantly neutrophils. H and E ×162. (b) At day 5, one may identify removal of necrotic muscle fibers, a process that continues for weeks until all or most of the necrotic fibers are removed. As the leukocyte infiltration begins at the periphery of the infarction, so does the removal of necrotic fibers. Courtesy from Dr. Juan Manivel and Mr. Dykoski Ricchard. Dept. Pathology. V.A. Hospital Minneapolis, MN. (c) In the third week following infarction, the stage of scar formation begins as a change in color of the depressed red band at the periphery of the infarction and the appearance of a ground- glass, gray hue. Histologically, it is represented by activity of fibroblasts and the formation of collagen fibers
Between 24 and 48 h, a yellow discoloration of the infarcted and non-infarcted areas is easily delineated. The gross feature corresponds to the established leukocytic infiltration into the infarction with a concentration at the periphery of the infarction.
At 2 and 3 days, the nuclei of the myocardial fibers tend to become indistinct and the cross-striations become coarse but do not disappear.
At day 5, one may identify removal of necrotic muscle fibers, a process which continues for weeks until all or most of the necrotic fibers are removed. As the leukocyte infiltration begins at the periphery of the infarction, so does the removal of necrotic fibers (◘ Fig. 4.4b).
In the second week, the picture of removal of myocardial fibers is characterized grossly by a band of reddish-purple between the yellow infarcted muscle and the normal muscle. Histologically, at this stage, there is fragmentation of muscle fibers and interstitial infiltration of macrophages and lymphocytes, while the stroma, composed of capillaries and supporting connective tissue, remains.
An irregular zone appears between the necrotic muscle of the infarction and the viable muscle. The zone is characterized by alteration between small masses of viable but ischemic muscle and small areas of infarction. This “junctional zone” may be termed “the twilight zone .” It may be responsible for the clinical complication of electrical failure after the acute episode and perhaps for the segmental and T wave changes characteristic of acute myocardial infarction.
In the third week following infarction, the stage of scar formation begins as a change in color of the depressed red band at the periphery of the infarction, now appearing as gray-hued ground glass. Histologically, it is represented by activity of fibroblasts and the formation of collagen fibers (◘ Fig. 4.4c).
By week 4, in most cases, the necrotic muscle is removed and its place is taken by scar tissue formed by the new connective tissue and the preexisting stroma . At the site, the cardiac wall is thinned, and the endocardium overlying the infarction increases in thickness (fibroelastosis).
Clinical Manifestations
The clinical diagnosis of myocardial infarction is generally justified on the basis of prolonged pain (1–2 h of severe anterior chest pain), but documentation requires QRS (Q wave) abnormalities shown on an electrocardiogram (ECG) as well as ST segment T changes and a rise in serum biomarkers that are released by the injured myocardium. While myocardial infarction may be without symptoms recognized by the patient (silent) or associated with typical symptoms, the majority of patients seek help because of unremitting chest distress with a few displaying perspiration, some dyspnea, and anxiety.
Deaths from ventricular arrhythmias are common in the first few hours if the patient is unattended.
Characteristically, patients experience mild fever and leukocytosis for 3–4 days, and a pericardial rub can be heard in many patients with STEMI.
Myocardial (pump) failure; rupture of the free wall, septum, or papillary muscle; and aneurysm formation are dreaded complications.
Postinfarction pericarditis is generally benign, but the severity of the distress and temperature elevation may be surprising, and cardiac tamponade can occur.
Complications of Acute Myocardial Infarction
Complications of acute myocardial infarction include mechanical (“pump failure”), arrhythmia, thromboembolism from the heart, and pericarditis.
A major arrhythmia may result in a sudden circulatory collapse. Mechanical failure usually means acute heart failure due to inadequate ventricular function and may be present with signs of acute pulmonary edema or shock, either alone or together.
Ventricular arrhythmia is a common complication of acute myocardial infarction, occurring in most patients experiencing an MI. It is related to formation of reentry circuits at the confluence of the necrotic and viable myocardium. Premature ventricular contractions (PVCs) occur in about 90 % of patients. The incidence of ventricular fibrillation is about 2–4 %.
The significance of ventricular fibrillation in myocardial infarction has been reevaluated in the context of the interaction between severe systolic dysfunction and the potential for sudden cardiac death. Although implantable defibrillators have reduced mortality in patients with an ejection fraction less than 30 %, regardless of the presence of ventricular dysrhythmia, their placement in the first month after MI has not proven beneficial. That results, in part, because many patients given prompt reperfusion have a significant recovery of LV function in that time period [21]. These patients also have a lower risk of serious dysrhythmias later on.
Supraventricular arrhythmias (mainly atrial fibrillation) occur in fewer than 10 % of patients with acute myocardial infarction. These patients tend to have more severe ventricular dysfunction and, potentially, a worse outcome.
Bradyarrhythmias, including atrioventricular (AV) block and sinus bradycardia, occur most frequently with inferior myocardial infarction. Complete AV block occurs in about 20 % of patients with right ventricular infarction. These episodes of heart block are usually associated with a narrow QRS complex; the site of conduction block is in the AV node. Infranodal conduction disturbances with wide, complex ventricular escape rhythms occur most often in large anterior myocardial infarction and portend a very poor prognosis.
Mechanical Complications
Mechanical complications of acute myocardial infarction include left ventricular failure and cardiogenic shock, cardiac rupture, and mitral insufficiency.
Left Ventricular Failure
The degree of LV pump failure is generally related to the size of the perfusion field distal to the thrombotic coronary occlusion. Infarction of more than 40 % of the LV muscle mass usually results in cardiogenic shock and death. Prior infarction, mitral insufficiency, an acquired left-to-right shunt (usually from an infarct-related rupture of the ventricular septum) [VSD], or a large aneurysm potentiates the effects of acute infarction on overall pump function, dramatically increasing the risk of death.
Other conditions not immediately related to the myocardial infarction, such as chronic pulmonary disease, non-infarct-related valvular heart disease, left ventricular hypertrophy, and hypertension, further increase the risk of heart failure and shock. Before reperfusion therapy was available, large infarcts often resulted in death due to low cardiac output or intractable arrhythmia. While these patients are still very ill, prompt reperfusion and temporary circulatory support can sometimes allow the muscle time to recover function and sustain life.
In instances where extensive myocardial infarction is responsible for LV failure, the infarction may be both acute and, in part, healed. When the infarction is only acute, LV failure usually means that the infarction is transmural and involves an extensive portion of the LV (◘ Fig. 4.5a–d).




Fig. 4.5
(a) Cross-sections of the ventricular portion of the heart. There is transmural acute myocardial infarction involving the anteroseptal area. (b) Echocardiogram in (Panel A) end diastole and (Panel B) end systole from a patient with STEMI demonstrating extensive anteroapical infarction with aneurysm (arrows), poor ejection fraction, and apical thrombus (T). (c) Panel A. T2-weighted scan shows bright segments of LV myocardium representing edema from an acute injury in the LAD distribution (thin arrows). Panel B. This scan demonstrates increased gadolinium uptake or delayed enhancement (arrows) in the left anterior descending (LAD) coronary artery distribution. The delayed enhancement is present in more than 50 % of myocardial wall thickness representing a transmural myocardial infarction (arrows). (d) Panel A. Cardiac magnetic resonance imaging (MRI) was performed 3 days after an occlusion of the left anterior descending artery. This scan shows the LV early after gadolinium. Microvascular obstruction or early hypoenhancement is present (thin arrows) from a lack of capillary filling. A large apical thrombus is also seen (fat arrow). Panel B. End-diastolic FFSP cineangiogram image of a patient following an acute anterior MI. The small arrow shows slow flow with an LV apical thrombus (Fat arrow)
Less commonly, the LV failure results from extensive, acute subendocardial infarction (circumferential infarction). When lesser amounts of acute myocardial infarction are present, LV failure may result from the acute infarction damage plus extensive damage from an old myocardial infarction. Preexisting diastolic noncompliance, much more common in women and patients with hypertension, doubles the risk of heart failure after MI. Conversely, patients with small, more distal infarctions may have discrete regional wall motion abnormalities with preserved overall LV function because of compensatory hyperkinesis of unaffected segments.
Congestive heart failure (CHF) occurs in about 15–25 % of patients who experience MI and is associated with an in-hospital mortality rate of 15–40 %. In their 2002 report based on the Second National Registry of Myocardial Infarction (NRMI-2), Wu et al. analyzed the outcomes for patients with ST elevation MI with CHF (Killip classes II and III), and they found that 19 % had CHF on admission [22].
Patients presenting with CHF were older and more often female and had a longer time to hospital presentation and a higher prevalence of anterior/septal AMI, diabetes, and hypertension. They also had longer lengths of stay and a greater risk for in-hospital death. Patients with CHF were less likely to receive aspirin, heparin, oral beta-blockers, fibrinolytics, or primary angioplasty and more likely to receive angiotensin-converting enzyme (ACE) inhibitors. Congestive heart failure on admission was one of the strongest predictors of in-hospital death.
Left Ventricular Failure and Cardiogenic Shock
Cardiogenic shock is defined as a state of inadequate tissue perfusion resulting from severe impairment of ventricular pump function in the presence of adequate intravascular volume. It is the leading cause of death for patients with acute myocardial infarction.
Despite advances in the treatment of myocardial infarction, the incidence of cardiogenic shock has remained at 7–10 % during the last 25 years. In a prospective study of 293,633 patients with ST elevation myocardial infarction (STEMI), Babaev et al. found that 8.6 % experienced cardiogenic shock [23].
Hospital mortality for patients experiencing cardiogenic shock was about 90 % in the 1970s. It has improved over the years, but in-hospital mortality is still estimated to be about 50 % [22]. For persons older than 75 years, the mortality rate is higher. The short-term survival rate has increased in recent years—at the same time that use of coronary reperfusion and temporary circulatory support strategies has increased.
The vast majority of patients with cardiogenic shock have LV failure ; in about 12 % of cases, cardiogenic shock is related to other infarct-related mechanical causes, including acute mitral insufficiency, ventricular septal rupture, and ventricular free wall rupture.
Risk factors for developing cardiogenic shock include STEMI, older age, anterior myocardial infarction location, hypertension, diabetes mellitus, multivessel coronary artery disease, prior MI, and prior CHF. All of these risk factors are correlated with either a large perfusion field distal to the thrombosed coronary lesion or to reduced diastolic compliance of the ventricle.
Most patients develop cardiogenic shock because of extensive myocardial ischemia or necrosis. This directly impairs myocardial contractility, resulting in diminished stroke volume and arterial pressure. On a mechanical level, a marked decrease in contractility, reduced ejection fraction and cardiac output, and, ultimately, ventricular failure result in systemic hypotension and/or pulmonary edema.
Varying pathological stages of infarction confirm the stuttering and progressive nature of myocardial necrosis. A combination of new and old infarctions consistently involves at least 40 % of the myocardium.
A systemic inflammatory response mechanism has been implicated in the pathophysiology of cardiogenic shock. Elevated levels of white blood cells, interleukins, and C-reactive proteins are often seen in large infarcts. Inflammatory nitric oxide synthase (iNOS) is also released in high levels and induces nitric oxide (NO) production, which may uncouple calcium metabolism in the myocardium, resulting in stunned myocardium. Additionally, iNOS leads to the expression of interleukins, which may themselves cause hypotension.
Elevated sympathetic neural tone and elevated circulating catecholamines increase systemic vascular resistance, cause pulmonary vein constriction, and may reduce blood flow to non-infarct-related coronary perfusion fields. The result can be a limitation of hyperemic blood flow to the remaining muscle, exhibiting compensatory hyperkinesis to make up for the loss of function in the infarct zone. All of these factors and the diminished coronary artery perfusion from hypotension trigger a vicious cycle of further myocardial ischemia and necrosis, resulting in even lower blood pressure, lactic acidosis, multiple organ failure, and, ultimately, death.
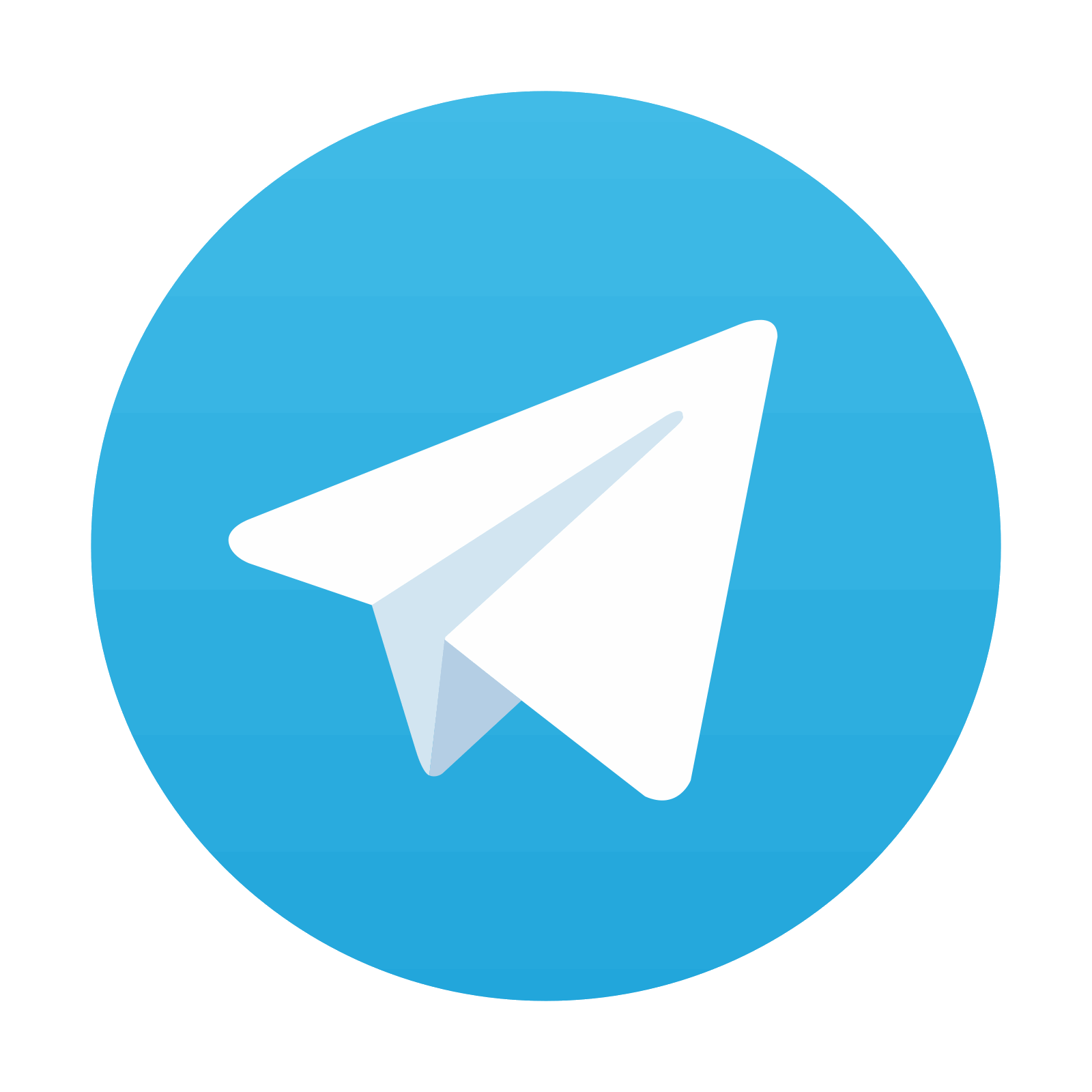
Stay updated, free articles. Join our Telegram channel
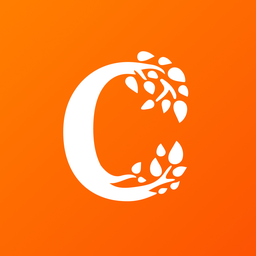
Full access? Get Clinical Tree
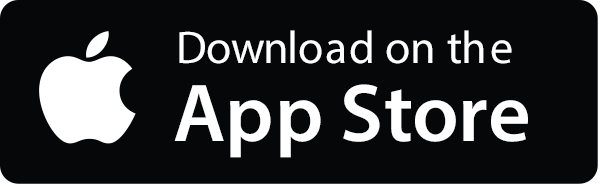
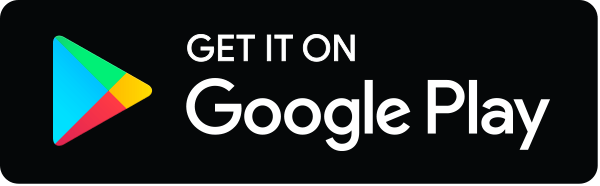