Fig. 3.1
Signaling pathways downstream of BMPR2
Interestingly, it is clear that not all persons with BMPR2 mutation develop PAH and within the same family there is variation in age of onset and disease severity. This variability suggests a “second hit,” perhaps an environmental exposure or additional genetic factor modulating risk of disease development [22]. Recent work has suggested that alterations in expression of the estrogen-metabolizing enzyme CYP1B1 favoring 16-hydroxyestrone over 2-methoxyestradiol may underlie development of PAH in many patients with BMPR2 mutation [23]. Alternative splicing of the normal BMPR2 allele in heterozygous mutation carriers may affect pulmonary vascular disease development [24].
Although BMPR2 mutation is found in many cases of HPAH and some sporadic PAH, we now know that BMPR2 expression is depressed in many forms of PAH including IPAH not associated with mutation [25]. Despite over a decade of research on BMPR2, the exact mechanism through which altered BMPR2 signaling results in the clinical phenotype of pulmonary vascular disease is unknown. Recent work has highlighted the potentially important role that cytoskeletal abnormalities as a function of rac1 defects and metabolic derangements regulated by BMPR2 signaling may play in PAH development [26]. Work continues to explore these and other hypotheses in the development of pulmonary vascular disease related to BMPR2 mutation and signaling depression. Downstream effects of BMPR2 signaling will follow later in this section.
Hereditary hemorrhagic telangiectasia (HHT) is another heritable vascular disorder linked to the TGF-β signaling pathway. Prior to discovery of BMPR2-associated PAH, mutations in two TGF-β signaling proteins (ALK-1 and endoglin) were demonstrated as the genetic basis of HHT [27–29]. Unlike its more common phenotype, a minority of subjects with HHT have PAH that is clinically indistinguishable and pathologically similar to those with IPAH [30, 31]. Mutations of endoglin or ALK-1 have been identified in those families affected by HHT and PAH [29, 31, 32]. PAH in children is often secondary to congenital heart disease, but when this is excluded, they may be more likely to carry an identifiable genetic mutation in BMPR2, endoglin, or ALK-1, reconfirming that different mutations in the TGF-β signaling pathway are often indistinguishable from one another and are frequently found in patients with idiopathic disease [33] (Table 3.1).
Table 3.1
Evidence for abnormal signaling pathways in PAH
Proposed signaling pathway | Evidence in cell culture | Evidence in animal models | Abnormal in human subjects | Therapeutic targets readily available |
---|---|---|---|---|
BMPR2 | Yes | Yes (genetic models) | Yes | No |
Smad | Yes | Yes | Yes | No |
MAPKK/MAPK | Yes | Yes | Yes | No |
LIMK | Yes | Yes | Lack of evidence | No |
Src | Yes | Yes | Limited data | No |
Nitric oxide | Yes | Yes | Yes | Yes, FDA approved |
Prostacyclin | Yes | Yes | Yes | Yes, FDA approved |
Endothelin-1 | Yes | Yes | Yes | Yes, FDA approved |
Serotonin | – | Yes | Yes (conflicting data) | Yes |
VEGF | Yes | Yes | Yes (conflicting data) | Yes |
PDGF | Yes | Yes | Yes (kinase inhibitors) | |
bFGF | Yes | No | Yes | Yes (kinase inhibitors) |
EGF | Yes | Yes | No | Yes (kinase inhibitors) |
Most recently, whole-exome sequencing was used in a family with multiple PAH-affected members in whom no identifiable mutation had been found. All affected family members, and some unaffected members, carried a mutation in the gene encoding caveolin-1 (cav–1), a protein important in formation of caveolae which are cell surface invaginations that modulate cell surface protein endocytosis and recycling. An additional sporadic case of IPAH secondary to cav–1 mutation was also discovered [34]. This study suggests that mutations in cav–1 are a rare cause of PAH and is the first mutation known that does not involve TGF-β signaling directly. Caveolin-1 is known to be important in vascular biology and physiology because it is a crucial negative regulator of eNOS activity [35, 36]. Recent studies using cav-1 and eNOS knockout mice suggest that the lack of cav-1 results in excessive eNOS activity associated with generation of ROS and inactivation of PKG (the major downstream target of NO-generated cGMP) by nitrosylation [37]. Cav-1 knockout mice develop cardiovascular disease including spontaneous PAH and RV failure when exposed to chronic hypoxia [38–40].
Vasoactive Substances and Imbalance
The hallmarks of pulmonary hypertension are elevated pulmonary arterial pressure (PAP) and vascular resistance (PVR). Patients with what is now categorized as IPAH were described early on in biopsy and autopsy series as those with normal lung parenchyma and pathologic findings limited to the small muscular arteries [41]. A logical area of investigation was the balance of vasoactive substances.
Vascular smooth muscle (SMCs) and endothelial cells (ECs) participate in regulation of blood flow in both the systemic and pulmonary vascular bed. The regulation of pulmonary circulation is unique to that of the systemic circulation especially in regard to hypoxic pulmonary vasoconstriction (see Chap. 4). While hypoxic pulmonary vasoconstriction is effective and necessary to maintain homeostasis in health and disease, chronic lung diseases featuring hypoxemia are a common cause of PH (WHO Group 3). While there is still debate as to the mechanism of hypoxic pulmonary vasoconstriction, research in this area has led to a better understanding of pulmonary physiology and a wealth of knowledge that has been adapted to the study of pulmonary vascular disease broadly.
It has long been known that histologic specimens from patients with PAH show normal lung parenchyma but intimal and medial thickening of the vascular wall and intraluminal findings of cellular proliferation and often thrombotic material [41–43]. In addition to pathologic findings limited to pulmonary vessels, an early observation was an acute response to administration of pulmonary vasodilators leading to the theory that the underlying pathobiologic mechanism was a “vasoconstrictive factor” [44–46].
Nitric Oxide
In the 1950s Paul Wood developed a classification scheme for PH not dissimilar to our current WHO classification. He was interested in vasoconstriction as a possible cause of elevated PAP and PVR. In a subject with mitral stenosis and PH, he administered acetylcholine, a known vasodilator. He noted that PAP and PVR fell and systemic pressure increased, presumably from increased cardiac output. This was shown to be reproducible in patients with PAH and PH secondary to mitral stenosis or COPD, but not in Eisenmenger’s syndrome [46]. Through elegant experiments in several labs over the ensuing three decades, acetylcholine’s effect was demonstrated to be via an endothelial dependent mechanism with the effector compound being nitric oxide (NO) from ECs and the target being the vascular SMC [47, 48]. Subsequently it was shown that strips of excised but still endothelialized PA from patients with Eisenmenger’s syndrome and chronic lung disease with secondary PH had blunted vasodilatory responses to acetylcholine, but preserved response to nitroprusside (an NO donor) [49, 50]. Endothelial dysfunction was proposed as the mechanism.
Nitric oxide is a short-lived powerful vasodilator produced from metabolism of L-arginine by the enzyme nitric oxide synthase (NOS). In the lung, endothelial NOS (eNOS) is the predominant isoform. Nitric oxide exerts its effect by increasing cyclic guanosine monophosphate (cGMP) within PASMCs which causes relaxation by activating cGMP-dependent protein kinase (PKG) that then acts on a variety of downstream targets to decrease intracellular calcium concentration. Inhaled NO reduces PAP and PVR in some subjects with PH [51]. Lung specimens from subjects with PAH show reduced or absent eNOS expression. Also, eNOS and endothelin-1 (a vasoconstrictor discussed below) have inverse staining patterns in the endothelium of PAH subjects compared with controls [52]. Subjects with PAH also have higher levels of ADMA (an eNOS inhibitor) and reduced expression of DDAH2 (an enzyme which metabolizes ADMA) in lung specimens compared to controls [53]. Taken together, these findings suggest that endothelial derived NO is reduced in PAH. Whether reduced NO availability is a cause or effect of pulmonary vascular disease is uncertain, but it has led to development of pharmacologic agents designed to increase pulmonary vascular cGMP levels. A family of phosphodiesterases (PDE) degrade cGMP and cAMP. PDE5 is the predominant isoform in pulmonary vascular smooth muscle and PDE5-specific inhibitors such as sildenafil and tadalafil have been shown to reduce PAP in animal models of pulmonary hypertension and in humans with PAH [54–56]. L-arginine has been used with limited success in the treatment of PAH and has been shown to reduce PAP and PVR in some subjects [57, 58]. Recently, soluble guanylate cyclase stimulators that act synergistically with NO to increase cGMP synthesis have also been approved for the treatment of PAH (see Chap. 15 for more discussion on cGMP modifiers for the treatment of PAH).
Prostacyclin
Prostacyclin is also produced by ECs and relaxes pulmonary and systemic smooth muscle [56]. Rubin administered prostacyclin to subjects with PAH and reported favorable acute hemodynamic changes [59] and later a favorable randomized trial of continuous IV prostacyclin therapy [60]. Despite the efficacious effects of prostacyclin (see Chap. 13), it was not known at that time whether abnormalities of prostacyclin synthesis contributed to the pathophysiology of PAH. Further studies found that thromboxane, that is derived from the same synthetic pathway as prostacyclin, but is a known procoagulant and vasoconstrictor, is increased in subjects with PH, whereas prostacyclin metabolites are decreased. The ratio of thromboxane:prostacyclin is increased in subjects with PH of any cause relative to controls [61]. This suggests increased platelet activation and lends further support to the presence of endothelial dysfunction in subjects with PH.
Endothelin-1
Endothelins (ET-1, ET-2, and ET-3) are another potent mediator of pulmonary vasoconstriction and are also SMC mitogens. Endothelin receptors ETA and ETB are both present in the pulmonary vasculature, although their relative expression varies depending on vessel size [62]. The action of ET-1 on ETA receptor on PASMCs causes vasoconstriction and mitogenesis. The ETB receptor has a less clear role in vascular tone, but plays an important role in clearance of ET-1. ETA receptor blockade in experimental conditions reliably attenuates endothelin-induced vascular SMC mitogenesis [62–66] and improves symptoms, exercise tolerance, and hemodynamic parameters in patients [67–71].
In vivo, plasma levels of ET-1 are higher in subjects with PAH or secondary PH compared to controls and are higher in the arterial than venous circulation [72, 73] suggesting increased production or reduced clearance within the pulmonary bed. Compared to controls, PAH subjects have increased ET-1 gene and protein expression in lung tissue which is concentrated in areas of vascular remodeling, especially plexiform lesions [74, 75]. Endothelin effects clearly contribute to pulmonary vascular disease but whether ET-1 overexpression initiates or only propagates pulmonary vascular disease is unknown. Likely, initial endothelial injury results in maladaptive ET-1 release which promotes mitogenesis of vascular SMCs and fibroblasts in patients already prone to uncontrolled cellular proliferation (i.e., those with BMPR2 mutation) or in those in whom endothelial injury is constantly present (i.e., congenital cardiac shunt). Regardless of mechanism, inhibition of the endothelin system has offered another attractive therapeutic target in the treatment of pulmonary hypertension (see Chap. 14).
Serotonin
The “serotonin hypothesis” of PAH was borne out of the observation that certain serotonergic medications are associated with PAH development [76–79]. Serotonin or 5-hydroxytryptamine (5-HT) causes intense vasoconstriction of systemic and pulmonary vessels and also acts as a mitogen for vascular SMCs [80, 81]. Within the circulation 5-HT exists almost exclusively within platelets for release “on demand,” and plasma levels are normally very low. After release from activated platelets, 5-HT is transported into cells including ECs and SMCs via the 5-HT transporter (SERT or 5-HTT) and metabolized by monoamine oxidase. 5-HT1B is the receptor most important for pulmonary vasoconstriction [82, 83].
Experimental disruptions of the 5-HT system have yielded some understanding of its role in human PAH but have not resulted in any therapeutic breakthroughs. Some studies have shown subjects with PAH to have higher concentrations of 5-HT in plasma and within platelets compared to controls which persist after lung transplantation [84]. Polymorphisms of the 5-HTT (the “LL” variant) are likely not associated with PAH in the largest and most recent studies [85–87]. PASMCs from subjects with PAH have higher expression of 5-HTT and are more susceptible to 5-HT-mediated mitogenesis which is reduced by 5-HTT inhibition [85]. Experimental overexpression of 5-HTT causes worsened PH phenotype in animals [88], and 5-HTT knockout mice are protected from pulmonary vascular disease [89]. There are various medications which target the 5-HTT with varying affinities, these being the selective serotonin reuptake inhibitors (SSRIs) commonly employed in the treatment of depression and anxiety. Proliferation of vascular SMCs in response to 5-HT in vitro is inhibited by SSRIs [85, 90]. SSRIs protect against PH in the chronic hypoxia mouse model [91]. In terms of receptors, it is known that the 5-HT1B receptor is involved in pulmonary vasoconstriction and sumatriptan, an FDA-approved medication for treatment of migraine and 5-HT1B/D receptor agonist, causes acute rise in PA pressure [83, 92]. Knockout of the 5-HT2B receptor and knockout or antagonism of the 5-HT1B receptor protect rodents from PH [93, 94]. Further study of the serotonin system and its role in pulmonary vascular disease is ongoing.
Solute Channels
Potassium channels regulate resting membrane potential and voltage-gated Ca2+ influx. Calcium is essential for both vascular SMC contraction and proliferation and higher cytosolic Ca2+ concentration leads to both. Subjects with IPAH have reduced PASMC expression and activity of voltage-gated KV channels [95, 96] which promotes membrane depolarization and Ca2+ influx. Resting and stimulated cytosolic Ca2+ concentrations are known to be higher in PASMCs from subjects with IPAH than controls [95, 97] and this is likely due to both the KV channel defect and upregulation of several Ca2+ channels and regulators [97–100].
Multiple circulating and autocrine vasoactive substances, some having mitogenic potential, are altered in subjects with PAH. The nitric oxide, endothelin, and prostacyclin systems have met realization of clinically useful pharmacologic targets. Further study of other vasoactive mediators may lead to additional treatment options which are sorely needed. There is substantial interaction of vasoconstriction and cellular proliferation which is discussed in the next section.
Cellular Proliferation and Vascular Remodeling
In addition to vasoconstriction, cellular proliferation is one of the major underlying commonalities of PAH pathogenesis. Pulmonary vascular remodeling and proliferation are manifested in all components of the vessel wall: media thickening with vascular smooth muscle cell (SMC) hypertrophy and proliferation, intimal thickening and fibrosis, and intraluminal obstruction of proliferative endothelial cells (ECs) and plexiform lesions. Regulation of the growth and differentiation of ECs and vascular SMCs has been studied extensively in health and disease. Multiple mediators are known, some affecting both ECs and SMCs. Several of these with a focus on their specific role in pulmonary vascular disease will be reviewed herein. Some small molecules, such as 5-HT and ET-1, with prominent pulmonary vasoconstriction properties which have already been discussed also modulate growth and differentiation.
Vascular Endothelial Growth Factor
Vascular remodeling with cellular proliferation is most apparent at plexiform lesions. These histologic lesions are unique to PAH (WHO Group 1). They are composed of proliferating and apoptosis-resistant ECs and other cellular components which have been debated to be myofibroblasts, SMCs, or undifferentiated mesenchymal cells [98]. Within plexiform lesions expression of vascular endothelial growth factor (VEGF), its receptor (VEGFR), HIF-1α, and other remodeling genes are increased [101–103]. VEGF is also increased in plasma samples from subjects with IPAH compared to controls [104]. While VEGF and VEGFR are required for normal angiogenesis, they are also an exploited pathway of malignant-transformed cells. Given increased expression of VEGF and VEGFR within plexiform lesions a cancer paradigm of PAH developed, suggesting that excessive angiogenesis due to a molecular defect within a clone of cells leads to the clinically manifest disease. Preclinical research on VEGF signaling and PH has given conflicting data and has not yet arrived at a therapeutic modality. Experimental overexpression of VEGF is protective in some PH models [105, 106]. Consistent with this, VEGFR inhibition via receptor blockade (with SU5416) in the chronic hypoxia model results in worsened PH and increased EC proliferation [107]. A different VEGF signaling inhibitor, the multikinase inhibitor sorafenib, reduces progression of established PH in monocrotaline-treated rats [108] and prevents PH development in rats exposed to chronic hypoxia and SU5416 [109]. Taken together, this indicates that VEGF signaling is important in vascular maintenance and interrupting the pathway can worsen pulmonary vascular disease phenotype. However, some downstream effects of VEGF signaling may ultimately be maladaptive. The complex relationship of VEGF signaling in pulmonary vascular disease animal models and varying results of treatment approaches has recently been reviewed [110]. Further study is needed in this intriguing area before clinical application can be developed for treatment of PAH.
Mitogens
Several mitogens have been evaluated in PAH including platelet-derived growth factor (PDGF), basic fibroblast growth factor (bFGF), and epidermal growth factor (EGF). While the precise function of PDGF in vivo is still somewhat of a question, it is known as a potent vascular SMC mitogen in vitro which also causes SMC and fibroblast migration. It has been implicated in the pathogenesis of multiple vascular and fibroproliferative diseases [111]. PDGF and its receptor (PDGFR) are increased in vessels of lung specimens from subjects with PAH [112, 113]. bFGF is another mitogen for ECs and vascular SMCs and has been found to be increased in patient samples [114] and overexpressed in patient-derived PA ECs. Interference of bFGF signaling via siRNA for bFGF reduces PASMC proliferation in vivo and in vitro [115]. EGF and TGF-α both activate EGFR and cause growth and vascular remodeling, a pathway exploited in some tumors and targeted pharmacologically. Overexpression of TGF-α leads to pulmonary vascular disease in mice through EGFR signaling [116], and EGFR is overexpressed in the monocrotaline PH model. Inhibition of this pathway is effective in vitro [117] and prevents development of monocrotaline-induced PH in rats, but is ineffective in a chronic hypoxia model [118]. In addition, EGFR expression is no different in clinical specimens of PAH patients versus controls [118]. EGF inhibition therefore has limited therapeutic promise.
Tyrosine Kinases
Many of the above substrates and receptors signal through tyrosine-kinase mechanisms. Multiple tyrosine-kinase inhibitors exist. One of the earliest, imatinib (Gleevec), has been studied in basic and clinical studies of PAH. Imatinib blocks PDGF-mediated PASMC proliferation. However, it does not mitigate the effects of many other known growth factors (VEGF, EGF, bFGF). In monocrotaline-treated or chronic hypoxic rats, imatinib improves pulmonary vascular disease via reduced downstream signaling of PDGF [113]. There are several clinical case reports of beneficial effect of treatment with imatinib (Gleevec) [119–121]. A small phase II study in PAH demonstrated a decline in PVR with imatinib compared to placebo [122]. A subsequent phase III study in PAH patients already on combination PAH therapy demonstrated improvement in 6-min walk distance and in PVR, but there was a greater incidence of hospitalization in the imatinib group and increased risk of cerebral hemorrhage in imatinib-treated patients who also were receiving warfarin therapy [123]. Other multi-targeted tyrosine kinase inhibitors have been considered in PAH, but predicting their clinical effects and avoiding off-target effects remain of concern. The importance of such considerations is exemplified by the finding that dasatinib can cause pulmonary hypertension and pleural effusions in patients being treated for malignancy [124]. These issues are discussed further in Chap. 17.
TGF-β Signaling
As discussed previously, mutations in the gene encoding BMPR2 are the genetic basis of disease in most cases of familial and many cases of sporadic PAH [1, 11, 12]. The TGF-β family of receptors including BMPR2 is highly evolutionarily conserved. BMPR2 is required for embryogenesis and alteration of signals downstream of BMPR2 result in defects in angiogenesis [125]. BMP signaling has been studied extensively since the discovery of a link between BMPR2 mutation and PAH. The major downstream pathways of BMPR2 include (1) Smad1/5/8 which signals through the common Smad2 transcription factor, (2) p38MAPK and Erk1/2, (3) Src, and (4) LIMK, among others. Smad 1/5/8 signaling is facilitated by cGMP activation of PKG. Activated PKGI binds to and phosphorylates BMPR2. In response to ligand binding, PKG1 detaches from BMPR2 and associates with activated Smads and then translocates to the nucleus where it regulates transcription as a nuclear cofactor for Smads [126]. Recent studies demonstrate that Smad signaling is impaired in mice with reduced PKG expression and activation of PKG by cGMP improves Smad signaling in a rat model of PAH [127].
The cellular effects of BMP stimulation and its disruption are dependent on cell type. It is helpful to review the effects of normal and abnormal BMP signaling in SMCs and ECs separately.
In proximal PASMCs, BMP inhibits proliferation, and promotes apoptosis and SMC phenotype differentiation [128–130]. Peripheral PASMCs may have a different response to BMP stimulation, responding with proliferation [130]. BMP’s effect on control of proliferation is mediated through Smad signaling [128, 130]. Subjects with IPAH have less Smad1 activation than controls and unchanged p38MAPK/Erk activation, resulting in an unopposed proliferative stimulus [128, 130].
In contrast to the predominant effect on PASMCs, BMPR2 activation in endothelial cells promotes survival, proliferation, and migration [131, 132]. This effect is also mediated through Smad signaling. BMPR2 gene silencing results in EC apoptosis and makes the endothelium susceptible to damage. In vivo, this loss of endothelial integrity may allow PASMCs to be exposed to serum growth factors and proliferate in the absence of BMPR2-mediated growth regulation. In addition, increased endothelial apoptosis may select for apoptosis-resistant clones which have been demonstrated in plexiform lesions of PAH subjects [98].
Notch Signaling
The Notch family of receptors are single transmembrane proteins which bind to ligands on the surface of adjacent cells. Upon this stimulus, the intracellular portion of Notch is cleaved and translocates to the nucleus where it acts as a transcription factor. Notch ligands are primarily expressed on ECs and the receptors allow cell-cell communication affecting vascular development [133]. There are four known Notch receptors (Notch1–4). Notch3 is expressed only in arterial vascular SMCs and is crucial for development of normal arterial morphology, as evidenced by the study of Notch3-null mice which lack a normal arterial muscular layer [134, 135]. Notch3 overexpression results in vascular SMC proliferation that continues post-confluence in vitro [136]. Li et al. [137] have studied the association of Notch3 and PAH in both humans and animal models, finding increased expression at an mRNA and protein level as well as increased HES-5, a downstream effector. Inhibition of this pathway is protective in the chronic hypoxic PH model. Notch is also known to interact with other pertinent signaling pathways including Smad1, HIF-1α, and VEGF [133, 138].
The components of the vessel wall are under constant stress and appropriate regulation of proliferation, migration, and apoptosis are required to maintain homeostasis in health and under insults of disease. Cellular regulation of these processes is complex and varies not only by cell type, but also by tissue bed. VEGF, VEGFR, BMPR2, and Notch are required for formation of normal vessels during development. When injury to the vascular wall occurs, autocrine and paracrine signals such as VEGF, ET-1, PDGF, EGF, 5-HT, and BMP (and likely many others) respond to direct cellular response. Dysregulation of these adaptive signals in PAH subjects leads to abnormal PASMC and EC proliferation. The number of PAH therapies that target these pathways is minimal. A greater molecular understanding of the intricate balance of this proproliferative/antiproliferative balance has been attained in the last two decades, but research is ongoing and translation to clinical care is still needed.
Inflammation and Infection
Within WHO Group 1, there is a subset of patients with associated inflammatory, immune, or infectious conditions known to result in pulmonary vascular disease [139]. The most common of these are the connective diseases (CTD) and infectious diseases HIV and schistosomiasis. The study of pulmonary vascular disease associated with these diseases has uncovered links with inflammation and immunity. At the same time, study of patients with IPAH and HPAH has also revealed elements of dysregulated inflammation. Thus, there appears to be a strong influence of the immune system on pulmonary vascular disease onset and progression.
Pulmonary hypertension is a frequent manifestation of multiple autoimmune conditions [139] with systemic sclerosis being the most common. Up to 12–40 % of patients with systemic sclerosis will develop PAH and this complication portends a poor prognosis [140, 141]. Systemic sclerosis and other CTDs are characterized by circulating autoantibodies, and clinical evaluation for these conditions is indicated in a patient with newly diagnosed PAH. Study of patients with IPAH has also revealed the presence of autoantibodies, primarily anti-Ku, which in one study was found in 23 % of presumed IPAH patients. Raynaud’s phenomenon, a common feature of systemic sclerosis, was frequently seen (39 %) in the same study [142].
Inflammation is a prominent feature of the pathology of pulmonary vascular disease. Pathologic review of several cohorts has demonstrated B- and T-lymphocytes (CD8+ and CD4+), macrophages, mast cells, and dendritic cells concentrated near areas of vascular remodeling [143–145]. Subjects with IPAH have increased circulating regulatory T-cells compared with subjects with CTEPH or normal controls but lower numbers of them in lung specimens [144–146]. There may also be alterations in natural killer (NK) cell population and function [147]. Any of these changes in circulating immune cell populations may result in altered cytokine response and inadequate control of immune activation manifested in the pulmonary vascular bed. In fact, multiple cytokines are known to be increased in PAH [148–150]. IL-6 levels are increased, correlate with clinical outcome, and do so better than hemodynamics or 6-min walk distance in some studies [149, 150]. Injection of IL-6 [151] or IL-6 overexpression [152] in mice results in PH, and knockout of IL-6 is protective against chronic hypoxic PH [153]. CX3CL1 (also known as fractalkine) and its soluble receptor (CX3CR1) are increased in the plasma and pulmonary endothelium of subjects with IPAH. CX3CL1 and CCL2 expression are elevated in plasma and in pulmonary ECs of PAH patients compared to controls. These chemokines increase leukocyte adhesion to ECs, further propagating perivascular inflammation. Increased cytokine production may be a central component of PAH but its temporal relation to disease development is uncertain.
Some infectious diseases are causally related to PAH development. In the USA, HIV is the most common, whereas schistosomiasis may be the most common cause of PAH worldwide. Up to 1 in 200 AIDS patients will develop PAH, meaning that HIV patients have up to a 600-fold higher incidence of PAH than the general population; [154, 155]. These patients have plexiform lesions indistinguishable from those seen in IPAH. However, the HIV virus has not itself been identified in pulmonary vessels [156, 157]. Indirect effects of HIV infection, either mediated by immune dysregulation or viral proteins, are proposed as mechanisms of pulmonary vascular disease. Several pertinent HIV proteins have been implicated, the most studied being the protein Nef. The predominant animal model of HIV, simian immunodeficiency virus (SIV)-infected macaques, has a low penetrance of pulmonary vascular disease. When infected with a chimeric HIV-SIV virus containing HIV Nef, they develop plexiform lesions containing Nef [158]. Nef, which has a soluble form, can enter non-HIV-infected cells via the chemokine receptor, CXCR4. In addition to lymphocytes, CXCR4 is present on ECs and may allow entry of Nef without direct infection of ECs by HIV virus [159]. Indeed, Nef has been demonstrated in pulmonary ECs of HIV-associated PAH subjects [150]. Nef increases IL-6 expression and multiple proproliferative signals including p38MAPK [155] which could promote PH development. More recently, study of the HIV envelope protein (Env) using SHIV-env in macaques also showed increased pulmonary vascular lesions in those animals compared to SIV-infected animals [160]. Exogenous Env protein has been shown to induce endothelial cell ET-1 expression. It is uncertain if this or another mechanism may underlie its role in HIV-associated PAH development. HIV proteins gp120 and Tat affect pulmonary ECs in vitro and could play a role, but data is preliminary [161, 162].
HHV-8 is a gamma-herpesvirus associated with Kaposi’s sarcoma which is a proliferative vascular lesion seen in patients with HIV infection. HHV-8 encodes a protein similar to IL-6 which induces VEGF expression explaining its disease manifestation. One group reported HHV-8 detected in lung specimens of 10 of 16 patients with IPAH and none of a matched secondary PAH cohort [163]. Since this study, multiple groups have failed to identify HHV-8 infection in various PAH cohorts [164–168]. As it stands, HHV-8 is felt to be unlikely to cause pulmonary vascular disease on its own.
Schistosomiasis is rare in the USA, but probably the most common cause of PAH worldwide. In chronic infections, endemic Schistosoma blood flukes reside in the mesenteric venous system and shed eggs and antigens causing an intense granulomatous reaction. This results in periportal fibrosis and portal hypertension, a syndrome known as hepatosplenic schistosomiasis [169, 170]. Hepatosplenic schistosomiasis precedes pulmonary vascular disease development, but the stepwise progression is uncertain. Regardless of cause, the histology is similar to that of other forms of PAH [171]. Mechanistic studies of PAH secondary to schistosomiasis are limited. One study of lung specimens from subjects with schistosomiasis and PAH failed to demonstrate the presence of egg antigens in pulmonary vessels, but it is uncertain whether antihelmenthic treatments may have eliminated their parasite burden [169, 171, 172]. Intense inflammation in this disorder may result in early pulmonary vascular changes and with continued immune activation, progression to an irreversible vasculopathy of the pulmonary bed. IL-13 has recently been implicated as a mediator of pulmonary vascular disease in schistosomiasis-associated PAH [173]. Schistosomiasis-associated PH is further discussed in Chap. 7.
Thrombosis and Hypercoagulability
Thrombosis has been a proposed central mediator or pulmonary vascular disease for decades. Pulmonary vascular thrombosis and thrombotic arteriopathy are common pathologic findings of PAH, found in 30–56 % of specimens [43, 174–176]. While incomplete evaluation for CTEPH in some studies may reduce the true incidence of thrombotic lesions, it is clear that many subjects with IPAH have some degree of pulmonary vascular thrombosis. Similar thrombotic lesions have been demonstrated in patients with congenital heart disease complicated by PAH, portopulmonary hypertension, and aminorex use [177]. These lesions are described as eccentric with post-thrombotic intimal fibrosis and recanalization. These changes are felt to be the same whether the cause was embolus or in situ thrombus formation [42, 178]. Much debate exists as to whether these real pathologic findings are an epiphenomenon of pulmonary vascular disease or rather a true contributor to disease development and/or progression.
In addition to circulating coagulation factors, the endothelium and its interaction with platelets are integral components of both prothrombotic and antithrombotic pathways [177]. While characterized inherited hypercoagulable states are no more common in patients with PAH or CTEPH than the general population [179], multiple studies demonstrate abnormalities in the prothrombotic and antithrombotic systems. In general, the pro/antithrombotic balance is tipped in favor of coagulation. Circulating von Willebrand factor (vWF) is increased in all forms of PAH [180], but its function may be reduced due to alterations of vWF multimer distribution [177, 180–182]. High shear stress in the pulmonary vasculature may contribute to depletion of high-molecular-weight multimers of vWF in PAH, akin to what occurs in the setting of left ventricular assist devices. vWF abnormalities due to endothelial dysfunction may promote platelet dysfunction and release of multiple mediators. For example, patients with IPAH have higher urinary thromboxane metabolites [61] and plasma 5-HT [83, 84]. Markers of thrombin action and fibrin formation (d-dimers, fibrinopeptide A, fibrin degradation products) are also elevated, but so are the anticoagulant/fibrinolytic factors such as thrombomodulin, tissue factor pathway inhibitor, and PAI-1, a fibrinolytic inhibitor [180, 183, 184].
Control of hemostasis is a complex, highly regulated system. The endothelium is a central component of this system and must balance both constant free-flowing blood and the possibility of endothelial disruption. Multiple lines of evidence suggest that pulmonary vascular disease, especially IPAH or HPAH, demonstrate multiple abnormalities of these systems which may lead to a procoagulant phenotype. Circumstantial evidence that anticoagulation may be beneficial in patients with PAH [185, 186] suggests that a procoagulant phenotype may at least play a role in continued or worsening pulmonary vascular disease.
Endocrine Factors
Rising rates of obesity and metabolic disease in the developed world coupled with recent registry data showing high rates of obesity in PAH [187] has brought intense interest into how endocrine factors may affect the pulmonary vasculature. The first observations were of high incidence of insulin resistance and glucose intolerance in subjects with PAH [188, 189], but other data has recently suggested that dyslipidemia may also play a role [190]. Animal models of PAH have been used to help define if these endocrine factors may play a role in disease promotion. Animal models of obesity, such as adiponectin-deficient mice and ApoE knockout mice, spontaneously develop PH [191, 192]. Moreover, pulmonary vascular disease is reversed by peroxisome proliferator activator receptor gamma (PPARγ) activation with rosiglitazone. BMPR2 signaling has been shown to be a key downregulator of PPARγ-mediated transcription and likely plays a role in promotion of insulin resistance in PAH [193]. Recently, we have demonstrated that in a model of HPAH using transgenic overexpression of mutant BMPR2, insulin resistance is present and worsened insulin resistance through a high-fat diet results in increased pulmonary vascular disease [194]. How insulin signaling is modified by BMPR2 mutation is currently under investigation, and it is presently unknown if reversing insulin resistance in humans with PAH may be a therapeutic option for this disease.
Other endocrine factors, such as estrogen signaling, are also implicated in PAH. The impressive female predominance in PAH has led to the hypothesis that estrogen may promote PAH development [2, 3, 195]. While there is extensive data in chronic hypoxic and monocrotaline models that estrogen and its metabolite 2-methoxyestradiol attenuate PH [196–200], these findings have not been replicated in human specimens or transgenic models of PAH. Indeed, there is growing evidence that estrogen may worsen pulmonary vascular disease in certain animal models that may more closely recapitulate human disease [80, 201–203]. At the same time, recent studies suggest that in patients with PAH, female sex is associated with better right ventricular ejection fraction, cardiac index, and pulmonary hemodynamics than male sex [204–206] and that estrogen and its metabolites may improve right ventricular function in healthy patients and those with cardiovascular diseases [207]. These findings may explain the better overall survival in women than in men with PAH [208]. Further study is needed to reconcile the potentially beneficial effects of estrogens in some animal models with detrimental effects in others and the female predominance of PAH in human disease.
Conclusion
In summary, there are many signaling cascades, exposures, and molecules implicated in PAH development and promotion. While advances have been made in developing successful drug therapy for this disease, none has resulted in a cure. Unfortunately, we do not understand the critical initiating event in PAH, nor how to interrupt it. Another unknown is whether human pulmonary vascular disease is reversible once established. These fundamental questions will need to be answered before curative or disease-altering therapy is available to patients with this devastating disease.
References
1.
Fessel JP, Loyd JE, Austin ED. The genetics of pulmonary arterial hypertension in the post-BMPR2 era. Pulm Circ. 2011;1(3):305–19. PubMed PMID: 22140620, Pubmed Central PMCID: PMC3224422.PubMedCentralPubMed
2.
Humbert M, Sitbon O, Chaouat A, Bertocchi M, Habib G, Gressin V, et al. Pulmonary arterial hypertension in France: results from a national registry. Am J Respir Crit Care Med. 2006;173(9):1023–30. PubMed PMID: 16456139.PubMed
3.
Badesch DB, Raskob GE, Elliott CG, Krichman AM, Farber HW, Frost AE, et al. Pulmonary arterial hypertension: baseline characteristics from the REVEAL Registry. Chest. 2010;137(2):376–87. PubMed PMID: 19837821.PubMed
4.
Larkin EK, Newman JH, Austin ED, Hemnes AR, Wheeler L, Robbins IM, et al. Longitudinal analysis casts doubt on the presence of genetic anticipation in heritable pulmonary arterial hypertension. Am J Respir Crit Care Med. 2012;186:892. PubMed PMID: 22923661.PubMedCentralPubMed
5.
Rich S, Dantzker DR, Ayres SM, Bergofsky EH, Brundage BH, Detre KM, et al. Primary pulmonary hypertension. A national prospective study. Ann Intern Med. 1987;107(2):216–23. PubMed PMID: 3605900.PubMed
6.
Loyd JE, Primm RK, Newman JH. Familial primary pulmonary hypertension: clinical patterns. Am Rev Respir Dis. 1984;129(1):194–7. PubMed PMID: 6703480.PubMed
7.
Morse JH, Jones AC, Barst RJ, Hodge SE, Wilhelmsen KC, Nygaard TG. Mapping of familial primary pulmonary hypertension locus (PPH1) to chromosome 2q31-q32. Circulation. 1997;95(12):2603–6. PubMed PMID: 9193425.PubMed
8.
Nichols WC, Koller DL, Slovis B, Foroud T, Terry VH, Arnold ND, et al. Localization of the gene for familial primary pulmonary hypertension to chromosome 2q31-32. Nat Genet. 1997;15(3):277–80. PubMed PMID: 9054941.PubMed
9.
Machado RD, Pauciulo MW, Fretwell N, Veal C, Thomson JR, Vilarino Guell C, et al. A physical and transcript map based upon refinement of the critical interval for PPH1, a gene for familial primary pulmonary hypertension. The International PPH Consortium. Genomics. 2000;68(2):220–8. PubMed PMID: 10964520.PubMed
10.
Deng Z, Haghighi F, Helleby L, Vanterpool K, Horn EM, Barst RJ, et al. Fine mapping of PPH1, a gene for familial primary pulmonary hypertension, to a 3-cM region on chromosome 2q33. Am J Respir Crit Care Med. 2000;161(3 Pt 1):1055–9. PubMed PMID: 10712363.PubMed
11.
Lane KB, Machado RD, Pauciulo MW, Thomson JR, Phillips 3rd JA, Loyd JE, et al. Heterozygous germline mutations in BMPR2, encoding a TGF-beta receptor, cause familial primary pulmonary hypertension. Nat Genet. 2000;26(1):81–4. PubMed PMID: 10973254.PubMed
12.
Deng Z, Morse JH, Slager SL, Cuervo N, Moore KJ, Venetos G, et al. Familial primary pulmonary hypertension (gene PPH1) is caused by mutations in the bone morphogenetic protein receptor-II gene. Am J Hum Genet. 2000;67(3):737–44. PubMed PMID: 10903931, Pubmed Central PMCID: PMC1287532.PubMedCentralPubMed
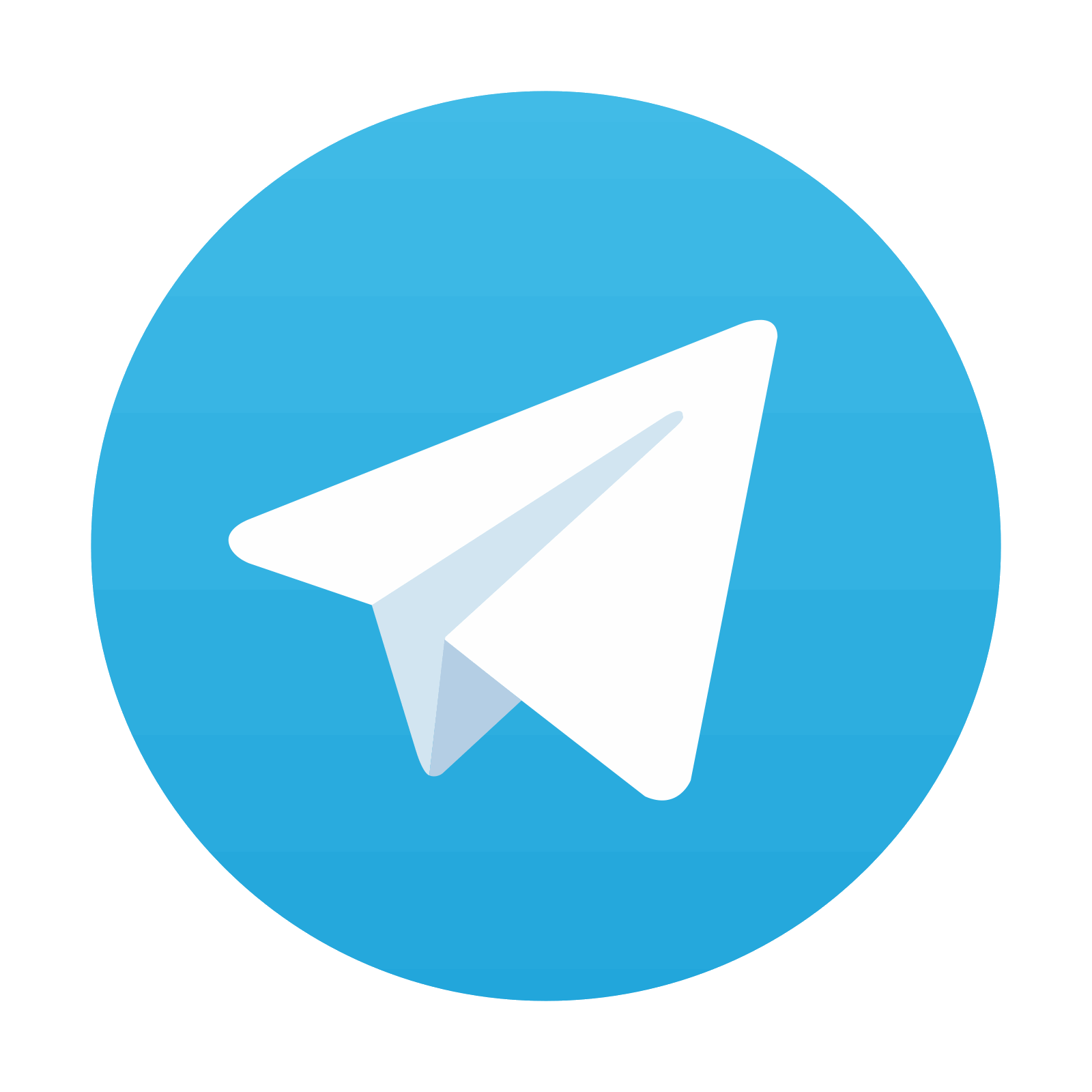
Stay updated, free articles. Join our Telegram channel
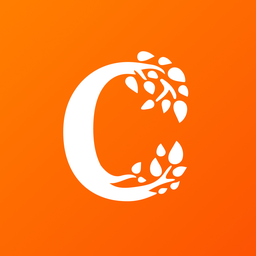
Full access? Get Clinical Tree
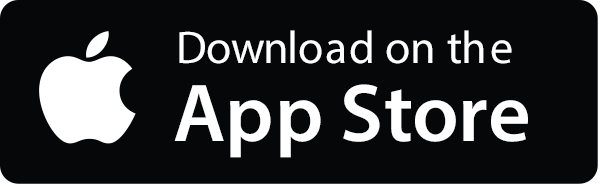
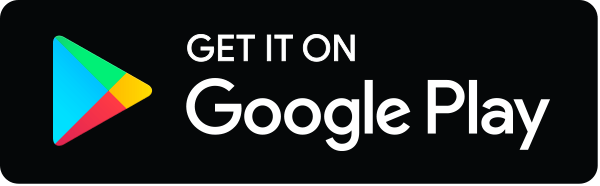